PROCEDURE_Crystal Violet Analysis by Spectrophotometry_Fall 2023
pdf
keyboard_arrow_up
School
Auburn University *
*We aren’t endorsed by this school
Course
CHEM 3051
Subject
Chemistry
Date
Dec 6, 2023
Type
Pages
15
Uploaded by aubreejordan13
CHEM 3051
Fall 2023 CRYSTAL VIOLET COLORIMETRY: A BEER'S LAW INVESTIGATION
LEARNING O B J E C T I V E S
The objectives of this exercise are to:
Illustrate the basic principles of colorimetry.
Demonstrate the components of a colorimeter and how a colorimeter is interfaced to a computer.
Discover the Beer's Law relationship and apply it to the analysis of an unknown solution. 1.0 BACKGROUND
Colorimetry is an instrumental method based on the measurement of light absorption by colored
solutions and is widely used for performing chemical analyses. The Micro
LAB colorimeter utilizes 10 Light Emitting Diodes (LEDs) ranging from 400 to 644 nanometers, spaced about 35 nanometers apart.
They are scanned sequentially and the transmittance or absorbance data is presented as 10 colored bars in
a bar graph as shown in Figure 1. Figure 1. MicroLAB spectrum profile for blank illustrating the color bars for each wavelength at 100 % transmittance
. These components are arranged such that light from each LED passes through the crystal violet solution
and falls on a photocell. The photocell circuit produces a current in microamps (I) which is
proportional to the light intensity striking the photocell surface and is converted into Transmittance and Absorbance by the MicroLAB software. These components are all housed in the colorimeter
chamber of the MicroLAB interface. Crystal violet (also called methyl violet) is an organic dye (See the reaction equation below). In crystal
violet solutions where the molarity of H
+ is greater than 0.1, the solution color is yellow. If H
+ molarity
less than 0.1, the solution color is purple. Our studies will involve purple solutions of the dye.
In this experiment you will prepare five crystal violet solutions of known concentrations varying from 2.00 x 10
-6 M
to 10.0 x 10
-6 M. The Transmittance for each of these standard solutions, for a water blank, and for a crystal violet solution of unknown concentration will be measured. The
Transmittance should decrease with increasing solution concentration while the Absorbance should
increase with increasing solution concentration. The Spectrum Profile for the absorbance of a CV solution is shown in Figure 2. Note that the maximum absorbance is at the 590 nm wavelength. Figure 2. Absorbance Spectrum Profile for CV. Maximum absorbance occurs at 590 nm.
.
One objective in this experiment is to find a mathematical function of Transmittance
that
is
directly proportional to sample concentration. Such a function does exist and forms the basis for what
is known as Beer's Law
. A graph of this function vs. concentration, which is linear and intersects the
origin, is called a Beer's Law plot. To explore this concentration/Transmittance relationship, you will first Hand Enter some "ideal" simulated data into the spreadsheet program. You will compute
and graph several current functions (the square, reciprocal and logarithm) until a function is that
has a direct proportionality to concentration is found. This function is then used for creating a Beer's Law plot from your actual experimental data. Finally, the concentration of the unknown crystal violet
solution is obtained directly from this plot. 1.1 Beer-Lambert Law in terms of Absorbance
Beer-Lambert Law, more commonly known as Beer's Law, states that the optical absorbance of a
chromophore in a transparent solvent varies linearly with both the sample cell path length and the
chromophore concentration. Beer's Law is the simple solution to describing the interaction of light
with matter. In practice, Beer's Law is accurate enough for a range of chromophores, solvents and
concentrations, and is a widely used relationship in quantitative spectroscopy. Absorbance is measured in a spectrophotometer by passing a collimated beam of light at wavelength λ
through a plane parallel slab of material that is normal to the beam. For liquids, the sample is held in an
optically flat, transparent container called a cuvette. Absorbance (A
λ
) is calculated from the ratio of light energy passing through the sample (I
0
) to the energy that is incident on the sample (I): A
λ
= -log(Transmittance) = -log (I/I
0
) Beer's Law follows: A
λ
= ε
λ
bc ε
λ
= molar absorptivity or extinction coefficient of the chromophore at wavelength λ
(the
optical density of a 1-cm thick sample of a 1 M solution). ε
λ
is a property of the material
and the solvent. b = sample path length in centimeters c = concentration of the compound in the sample, in molarity (mol L
-1
)
Your preview ends here
Eager to read complete document? Join bartleby learn and gain access to the full version
- Access to all documents
- Unlimited textbook solutions
- 24/7 expert homework help
Note the subscript in the absorbance factor, A in the Beer’s
law equation. The absorbance of a
solution depends on the wavelength or color of the incident light. Substances do not absorb the same
amount of light at all wavelengths. At specific wavelengths absorbance reaches a maximum and this
particular wavelength is max
. All measurements of concentrations must be made at this wavelength. In an absorbance experiment, light is attenuated not only by the chromophore, but also by reflections
from the interface between air and the sample, the sample and the cuvette, and absorbance by the
solvent. These factors can be quantified separately, but are often removed by defining I
0 as the light
passing through a sample "blank" or "baseline" or reference sample (for example, a cuvette filled with
solvent but zero concentration of the chromophore is used as the blank). Many factors can affect the validity of Beer's Law. It is usual to check for the linearity of Beer's Law
for a chromophore by measuring the absorbance of a series of standards. This "calibration" can also
remove errors in the experiment, the equipment, and the batch of reagents (such as cuvettes of unknown path length). 2.0 SAFETY PRECAUTIONS
Crystal violet solutions may cause skin and eye irritation. Wear safety goggles at all times. Wear gloves whenever handling chemicals including water. As usual, wash hands with soap and water before leaving the laboratory. Crystal violet stains, so appropriate care should be taken in its handling. 3.0
EXPERIMENTAL PROCEDURE
3.1
Simulated concentration/current data treatment
1.
Open Microsoft EXCEL
and enter the following "ideal" simulated data
into two columns. Use Column A for the concentration values and label as Molarity. Use Column B for the current values and label as Current (I). Concentration (M)
Current (I)
0.00 x 10
-6
600.0 2.00 x 10
-6
476.4 4.00 x 10
-6
378.6 6.00 x 10
-6
300.6 2.
After entering the data, generate an x-y scatter plot with transmittance on the y-axis and concentration on the x-axis
. Right-click on any data point and select Add Trendline
. Choose a Linear Curve fit as the type of plot desired, and EXCEL
will fit the data with the best possible straight line. Select the boxes to “Display Equation on chart” and “Display R
-
squared value on chart”. Note in this case, the data points would be better fit by a curved line, and hence, the correlation (Corr.) is poor. An acceptable Correlation value for a linear plot would be at least 0.999. Print this graph for your report.
3.
In separate columns on the spreadsheet, transform the transmittance data to I
2
, 1/I, and Log(I). Make a separate plot using each of these values on the y-axis, and concentration on the x-axis. When you discover the linear plot, leave the graph on the screen and wait until
all groups arrive at the same point. Your instructor will then discuss the modifications needed to give a straight line with a positive slope which also passes through the origin
. 4.
Make notes on the development of Beer's Law in your notebook. 3.2
Solution Preparation
A stock 10.0 x 10
-6 M crystal violet (CV) solution is provided. Perform dilution calculations to complete
the following table (see your text if needed) and have your work checked for correctness by your lab instructor. Dilution Table for Standards
CV Std #1
CV Std #2
CV Std #3
CV Std #4
CV Std #5
Desired CV Molarity
2.00 x 10
-6
4.00 x 10
-6
6.00 x 10
-6
8.00 x 10
-6
10.00 x 10
-6
mL CV
Total mL
50.00
50.00
50.00
50.00
50.00
Absorbance
Transmittance
Use graduated cylinders, pipets, or burets to transfer the CV solution volumes calculated in the above table into a series of dry 50 mL volumetric flasks. Use great care in your measurements, as careless work will be rewarded with unacceptable
Beer's Law plots and the necessity of repeating the experiment. Add deionized water to the mark. When all five solutions have been
prepared, stopper or parafilm the flasks and mix by gently inverting them several times to avoid bubbles. Label each solution according to the above numbering. 3.3
Measurements
5.
Select Spectrophotometer Experiment from the Main MicroLAB Menu to carry out the
experiment. Your data will come from the MicroLAB Interface. 6.
Your first sample should be the water blank (0.00 M
). Fill the colorimeter vial
about three-
fourths full, wipe the outside faces with a KimWipe, insert the vial into the
colorimeter and place the light cover over the vial
. The
program will automatically shift to Read Knowns after the Blank is read. 7.
For each sample of CV, rinse the vial three times with small amounts of the solution, then fill
the vial three-fourths full, insert it into the colorimeter, place the cap over the vial and click
the ADD button.
8.
The program will direct you to enter the concentration. It will then display the transmittance
for that sample. Follow this procedure for each of the standard solutions in order of increasing concentration. Be sure to wipe the vial with a KimWipe each time. 9.
Although the software records the transmittance and absorbance, note these quantities and
concentrations in your notebook also. Make a table of concentration vs. absorbance and
transmittance. 10.
For your unknown solution, click on Read Unknowns and measure the transmittance in
the same manner as was done for the standards. Note the Unknown letter in your notebook
. 11.
Using the digital calipers, determine the path length of one of your sample vials. Record this value in your notebook
. 4.0
CLEAN UP 12.
Pour all crystal violet solutions into the designated waste container. DO NOT POUR ANYTHING DOWN THE SINK!
13.
Discard empty vials in the broken glass box. 14.
Wash all glassware with detergent and place above sink to dry. 15.
Exit MicroLAB
software, and shut down the computer. 5.0
DATA ANALYSIS
16.
Obtain printouts of: •
Each of your Hand Entered data graphs, as modified by EXCEL
for each
mathematical transformation. •
The dilution table used to make your CV solutions. •
Your table of absorbance/trasmittance values for each CV and unknown solution (from MicroLAB). •
An example spectrum profile (from MicroLAB). •
Your Beer's Law plot made in EXCEL with your data. 17.
Use your Beer's Law plot regression equation to calculate the concentration of the unknown, and then compare it against the value that was given in MicroLAB. Show your calculations for the concentration of your unknown. As discussed by your instructor, Beer's Law is expressed by the equation A =
bc. In this equation, A is the absorbance of the solution, b is the cell path length (measured with calipers), c is
the molar concentration of the light-absorbing solute (CV), and, is a proportionality constant
called the molar absorptivity. 18.
Calculate the molar absorptivity for each of the five standard CV solutions and determine the average and standard deviation of the five values. Watch significant figures and be sure to
include proper units. Note how well your values agree with one another. After all, it is
supposed to be a constant!
Your preview ends here
Eager to read complete document? Join bartleby learn and gain access to the full version
- Access to all documents
- Unlimited textbook solutions
- 24/7 expert homework help
19.
Compare the numerical value of your average molar absorptivity with the slope of the best
straight line given on your Beer's Law plot. 6.0
REPORT
Prepare a title page and abstract according to the format detailed in the syllabus. Complete and include the calculations and data tables as instructed in Section 5.0 above. Also include in the report answers to the questions/problems listed below in Section 7.0. 7.0
Questions and Problems
1.
When iron (III) chloride is added to a solution of salicylic acid a purple solution forms. The photocell current
read with the colorimeter for a series of standard solutions is given below. Convert these values to absorbencies.
(The blank current is 100.0.) FeCl3
Transmittance
Absorbance
40.0 x 10
-5 M 17.9 32.0 x 10
-5 M 25.0 24.0 x 10
-5 M 35.7 16.0 x 10
-5 M 50.2 8.0 x 10
-5 M 70.8 2.
What would be the effect of the following on the Beer's Law plot? a.
At the higher concentrations used to construct the plot, some solute molecules react with one
another to form a species that doesn't absorb visible light. b.
A fingerprint is present on the solution cell where the light beam passes through. c.
Some solute molecules react with one another to form a species that doesn't absorb visible light.
Additional Reading
Dye Structure and Color
What dyes are: In short, dyes are colored, ionizing, aromatic organic compounds. It must be appreciated that they are individual chemicals, and like all chemicals, they are similar in
their reactions to some other chemicals, and distinctly different from others. It may seem that this is a
statement of the obvious, but we sometimes appear to view dyes as something other than ordinary
chemicals, and I want to stress that the same rules that apply to sodium chloride, acetic acid, benzidine
and a host of others also apply to dyes. This includes the possibility that they are toxic. They may be
carcinogenic or mutagenic, or harmful to your health in some other way. Just because we call something by an appealing name and because it has an appealing color, does not
make it any the less harmful. Handle dyes with care! Put your own safety first! What color is: Dyes are aromatic organic compounds, and as such are based fundamentally on the
structure of benzene. To us, benzene appears to be a colorless fluid. In fact it absorbs electromagnetic
radiation just as dyes do, but it does so at about 200 nm so that we do not see it. The perception of color is an ability of some animals, including humans, to detect some wavelengths of electromagnetic radiation (light) differently from other wavelengths. Normal daylight, or white light, is
a mixture of all the wavelengths to which we can respond and some to which we cannot, in particular
the infra-red and ultra-violet rays. We respond to wavelengths between about 400-700 nm. When an
object absorbs some of the radiation from within that range we see the waves that are left over, and the
object appears colored. In reality this range we see makes up only a very small fraction of the
electromagnetic spectrum. In scientific terms there is nothing special about the wavelengths in the visible range, other than being
the major components of sunlight which are not removed by the earth's atmosphere. Their special
importance is based exclusively on the ability of human retinas to respond to them, and to discriminate
between them to a significant degree. These discriminations are what we call color. Wavelengths just outside the visible range are considered colorless, even though there is no substantive
difference between them and the limiting wavelengths inside the range. Some animals (bees, for example) can see these other wavelengths but, because humans do not, we consider them colorless.
The point is that color is a subjective phenomenon, and thinking of color as something objective is
misleading. For that reason, we should refer to the wavelengths involved rather than describe the
human response to them.
When some of the wavelengths found in white light are absorbed, then we see what is left over as colored light. The color that we see is referred to as the complementary color of the color that was removed. For instance, if the red rays are removed from white light, the color we detect is blue-green.
Blue-green is complementary to red, and red is complementary to blue-green. Complementary Colors
Removed Observed
Removed Observed
Yellow-green Violet Violet Yellow-green Yellow Blue Blue Yellow Orange Cyan Cyan Orange Red Blue-green Blue-green Red Purple Green Green Purple Absorption of light leaving the complementary color The perception of color is merely a human evolutionary adaptation to the absence of some wavelengths
in white light. Suppose however, that the same thing happens outside the range to which our eyes
respond. Suppose a chemical removes radiation which has a wavelength about 200 nm, as benzene
does. Is this colored? Well, obviously it is not. There has been no impact on the wavelengths of white light, so it is not
colored. However, what has happened to the ultra-violet radiation that was absorbed by the benzene is
no different to what happens to the blue radiation that is removed when we look through a solution of acid fuchsin. The difference is solely whether we can detect it visually. Color is not an objective
phenomenon; it is the human detection and perception of electromagnetic radiation. Why dyes are colored
Color in dyes is invariably explained as a consequence of the presence of a Chromophore
. Since, by definition, dyes are aromatic compounds their structure includes aryl rings which have delocalized
electron systems. These are responsible for the absorption of electromagnetic radiation of varying
wavelengths, depending on the energy of the electron clouds. For this reason, chromophores do not
make dyes colored in the sense that they confer on them the ability to absorb radiation. Rather,
chromophores function by altering the energy in the delocalized electron cloud of the dye, and this
Your preview ends here
Eager to read complete document? Join bartleby learn and gain access to the full version
- Access to all documents
- Unlimited textbook solutions
- 24/7 expert homework help
alteration results in the compound absorbing radiation from within the visible range instead of outside
it. Our eyes detect that absorption, and respond to the lack of a complete range of wavelengths by seeing color. Chromophores are atomic configurations which can alter the energy in delocalized systems. They are
composed of atoms joined in a sequence composed of alternating single and double bonds. Double
bonds in organic compounds can be of two types. If the atoms with double bonds are not adjacent, they
are termed isolated double bonds, and exist independently of other double bonds in the same molecule.
If adjacent atoms have double bonds they are termed conjugated double bonds and the bonds interact
with each other. Chromophore configurations often exist as multiple units, having conjugated double
bonds, and are more effective when they do so. This is due to the interaction between the double
bonds, which causes partial delocalization of the electrons involved in the bonds. In this case, although
specific atoms are involved in the bonds, the electrons are distributed over a larger area than the
specific atoms and also involve adjacent atoms that have double bonds. The point of this is that conjugated systems have partially delocalized electrons, and the energy in
these delocalized electrons can impact on the energy of the delocalized electrons of the parent aromatic
compound by extending the number of electrons involved in the system and the energy needed to keep
the whole system in place. Conjugated double bonds (red) Another common chromophore is the nitro group. This chromophore is a nitrogen with two oxygen
atoms attached. One oxygen is shown attached with a single bond, the other with a double bond. In fact, like the carbon atoms in benzene, these two oxygen atoms are attached to the nitrogen with bonds of equal strength. The extra electrons are delocalized between the three atoms. Nitro group The quinoid ring is found in many dyes. It is a ring structure with two points at which chromophores can attach. It should be thought of as a closed system of conjugated double bonds. The attachment of configurations which add delocalized electrons to the system at one point, and the attachment of configurations which extend the atoms involved in the delocalization at the other, causes very dramatic
shifts in the wavelengths which these compounds absorb. For this reason, quinoid ring configurations
are considered to be extremely powerful chromophores, producing very intensely colored compounds. Ortho- and para- quinoid ring chromophores To sum this up, chromophores are atomic configurations that contain delocalized electrons. Usually
they are represented as nitrogen, carbon, oxygen and sulfur that have alternate single and double
bonds. By incorporating the delocalized electrons in these configurations into the delocalized electrons
in the aryl rings of aromatic compounds, the energy contained in the electron cloud can be modified. If the energy incorporated into the electron cloud is changed, then the wavelength of the radiation it
absorbs will also change. If this change in the wavelength to be absorbed is sufficient to cause any
absorption at all within the visible range, then the compound will be colored. Below are the usual chromophores seen in histological dyes:
–
–
C=C
–
–
C=N
–
–
C=O
–
–
N=N
–
–
NO
2 Quinoid rings Other effects
There is more than one effect when chemical groups are attached to aryl rings. Any delocalized
electrons and their energy can simply be added to that already present, thus increasing it. Also, the
delocalized electrons may be shared by more atoms than those in the original structure, by adding in to
the delocalized system the atoms in the chromophore and any modifiers that may be present. Whether
these effects occur jointly or separately, the final impact is an alteration in the overall energy of the
electron cloud with a subsequent effect on the wavelength of radiation to which the whole molecular
system reacts. Another possibility is that electrons may be removed from the electron cloud, and this may result in
loss of color. Removal of electrons may cause the remaining electrons to revert to local orbits. A good example would be Schiff's reagent. When sulfurous acid reacts with pararosanilin, a sulfonic group attaches to the central carbon atom of the compound. This disrupts the conjugated double bond system
of the quinoid ring, causing the electrons to become localized and the ring to cease being a
chromophore. Consequently, the dye becomes colorless. Auxochrome
Below are the usual auxochromes found in histological dyes:
–
–
NH
3 –
COOH –
HSO
3 –
OH Auxochromes are groups which attach to non-ionizing compounds yet retain their ability to ionize.
While this definition is largely correct, it is also inadequate. This is because it restricts the definition of the auxochrome to ionization, and does not comment on the effect of auxochromes on the absorbance
of the resulting compound. The word auxochrome is derived from two roots. The prefix
auxo is from auxein
, and means increased. The second part, chrome means color, so the basic meaning of the word auxochrome is
color increaser. This word was coined because it was noted originally that the addition of ionizing
groups resulted in a deepening and intensifying of the color of compounds.
Color enhancing by an auxochrome
To the left is naphthalene
, a colorless compound. The addition of a single hydroxyl group to naphthalene
produces 1-naphthol which is also a colorless
compound, but one which can ionize. If instead of a hydroxyl group we add the nitro group, which is a chromophore, we get the compound 2,4- dinitronaphthalene
. The addition of this chromophore
has caused it to become pale yellow. If instead of a hydroxyl or nitro groups, both a hydroxyl
and nitro groups are added, we get the deep yellow dye,
martius yellow
. The addition of both an auxochrome and a chromophore results in a much
stronger alteration of the absorption maximum of the compound. The
hydroxyl group must have deepened the color, showing that auxochromes are also chromophores. Sometimes the term auxochromophoric is used to denote the action of an auxochrome that modifies
the color as well as permitting ionization. This term infers that the color modifying effects of auxochromes are rare, but this is not the case. The effect on absorption should not be considered an
incidental aspect of the auxochrome's action, but an integral and fundamental part of it. The word auxochromophoric is redundant. Edward Gurr proposed the terms colligator and non-colligator to distinguish between ionizing
auxochromes and color modifying effects. Auxochromes are of two types. They may have a positive charge as the amino group and its substituted
variants. Or they may be negatively charged as the carboxyl and hydroxyl groups, and the sulfonic
group. This last is commonly used to convert basic dyes to acid dyes. Both negatively charged and
positively charged auxochromes may be present on a single molecule. Resonance
The process by which electrons are stimulated by radiation is resonance
. It should be made clear at the
outset that resonance is not the same as vibration. Resonance is the induction of a response in one energy system from another energy system in close
proximity, which is operating at the same energy level (frequency). In aromatic organic compounds,
Your preview ends here
Eager to read complete document? Join bartleby learn and gain access to the full version
- Access to all documents
- Unlimited textbook solutions
- 24/7 expert homework help
including dyes, the two energy systems are electromagnetic radiation
, and the delocalized electron cloud
. Do not confuse resonance with the imaginary resonance hybrids used in an older explanation
for the structure of benzene. Modifiers
Absorption of benzene compared to some dyes Color modifiers such as methyl or ethyl groups alter the color of dyes by altering the energy in the
delocalized electrons. By themselves they cannot do this enough to cause absorption in the visible
range, but they can affect the shade significantly when absorption is already in that range. Adding more of a particular modifier results in a progressive alteration of color. Compounds that differ from
each other in this kind of regular fashion are called homologues
. A very good example is seen with the
Methyl violet series. Alteration of color by modifiers
Without any methyl groups the
parent dye is called
pararosanilin and is red. When four methyl groups are
added we get the reddish purple
dye methyl violet
. As more methyl groups are
added we get the purple blue dye
crystal violet which has six such groups.
The Color
The color of the dye is caused by the absorbance of electromagnetic radiation. We have constantly
referred to the wavelength that is absorbed in the singular, but a simple scan of a dye solution with a
spectrophotometer shows that dyes do not remove a single wavelength. Rather they absorb radiation on either side of the wavelength most completely removed (the absorption maximum). Plotting the
wavelength absorbed against the degree of absorbance usually results in a display resembling a bell
curve. If any part of this curve is in the visible range, the dye will appear colored. White light is a mixture of wavelengths. Some of these have a relationship to the energy in the
delocalized electron cloud of the dye molecule. By the process of resonance, previously described, the
electron cloud will respond to the energy contained in that radiation by absorbing it, and removing it
from the spectrum. As a consequence the white light will cease to be white and will display the colors of the wavelengths left over. The transmitted light will have the complementary color to the
wavelengths removed. The Effect
When light illuminates a dye, some of it is absorbed as energy. Since energy is not destroyed,
something must then happen. We could use an analogy of heating water - the water's temperature rises, molecular vibration increases. However, we do not see anything else, as the water just sits there being
water. The same can happen with dyes, we may not observe anything particular as the effect may be at the
atomic level. There are several possibilities, however. A.
The energy level in the electrons in an unaffected dye is called the ground state. When
electromagnetic radiation, as light energy, is absorbed the electrons become more energized. B.
With most dyes, there is then a gradual decay and the electrons return to the ground state. We
do not see anything. Nevertheless, something may happen that we do not see. Possibly there is
an increase in temperature, or some chemical changes occur which disrupt the dye's structure
and cause it to lose color - fading
. C.
Another possibility is that the return to the ground state is not gradual, but sudden. If this is
accompanied by emission of any residual energy in the form of light, we observe the dye
glowing - fluorescence
. Since the emitted light must always contain less energy than the
absorbed light, as some was used to energize the electrons, the emitted radiation is always at
longer wavelengths than the absorbed radiation. By manipulating the light available, we can
cause ultra-violet light to be absorbed and visible light to be emitted. If a seventh methyl group is
added, the resulting dye is
methyl green
.
D.
A third possibility is that the electrons stabilize in their newly energized state. After a passage
of time they then return to the ground state. If this happens gradually, we may observe nothing,
with the same possibilities regarding fading and temperature increase as before. E.
If return to the ground state happens suddenly, and the residual energy is emitted as light, we once again see the dye glowing - phosphorescence
. As with fluorescence, the light emitted is
always a longer wavelength than the light absorbed, but the disparity is greater with
phosphorescence due to the greater energy consumed in keeping the electrons in the excited
state. Note that the difference between fluorescence and phosphorescence is in whether the electrons
stabilize in the excited state before returning to the ground state. With any stability, no matter how long (or short), it is considered phosphorescence. Conclusion
The explanation of the relationship between structure and color depends on the basic atomic structure
of the aryl ring, and the shared or delocalized electrons that this atomic arrangement has. The ability to
absorb radiation is inherent in this structure. The effect of other atomic configurations is to modify the
energy contained in the delocalized electron cloud so that the compound absorbs electromagnetic
radiation at a wavelength in the visible range. Some also ionize, enabling the compound to chemically
react with ionizing tissue groups. Color, fading, fluorescence and phosphorescence are all seen to be different effects of the same
fundamental process.
Your preview ends here
Eager to read complete document? Join bartleby learn and gain access to the full version
- Access to all documents
- Unlimited textbook solutions
- 24/7 expert homework help
Related Documents
Related Questions
7. Don believes that fewer than one-third of the students at his college
hold part-time jobs while in school. To investigate this, he
randomly surveyed 116 students and found that 32 students held
part-time jobs.
a. ( - Provide a 90% confidence interval for the population
proportion of students that hold a part-time job.
b. 1
(a).
;Give the correct interpretation of the interval in part
c. Extra Credit: Does this interval support Don's claim? Why?
arrow_forward
20,18,2,4,15,15,10
Using these data, construct a 80% confidence interval for the average net change in a student's score after completing the course. Assume the population is approximately normal.
Construct the 80% confidence interval. Round your answer to one decimal place.
arrow_forward
HP TrueVision HD
4 Texas Workfor
Mộc Homework ×
W. College Physic
mical App
AN Careers
eagleonline.hccs.edu/courses/188795/assignments/3601808
valnabie diler reD 4 dl 10.1Tam
Constants | Periodic Table
Part O
Complete the fourth row.
Complete the following table by calculating the
missing entries and indicating whether the solution
is acidic or basic.
Express your answer using two decimal
places.
Acidic or
[H+]
[OH-]
pH pOH
basic?
7.4x10-3
ΑΣφ
3.3x10-10
M
pH
8.25
5.73
Is
to
fa
ho
II
10
insert
arrow_forward
1.
Data & Results
4.0 mglmL
Concentration of stock solution (include units):
Table 1-Beer's Law Plot Data
V Volume of standard
solution dispensed (mL)
M, Diluted concentration
(include units)
Sample
Absorbance
A
2.00 mL
0.084
4.00 mL
0.173
l6.00 mL
0.259
8.00 mL
0.340
E
10.00 mL
0.418
Equation of trendline from Beer's Law plot:
whats the diluted concentration and equation of
trendline from the beers law plot once created?
arrow_forward
i need help calculating the molar concentration for part C
arrow_forward
I need help. Using a standard
curve: Another student produced
the standard curve shown here (
Fig.2.2) which shows the linear
relationship between
absorbance and Cu2+
concentration. They then
measured the absorbance of
their unknown solution to be
0.48126 AU. Use their trendline
equation to calculate the
concentration (M) of Cu + in their
Unknown Solution. Show your
work and consider sig figs.
arrow_forward
Determine the standard concentration of A through E and then the absorbance. I just need clarification on if I add together the two ml values given.
arrow_forward
Table 1. pH of water samples
Sample No.
Water Samples
pH
1
Tap water from household
6.59
2
Water from creek
7.35
3
Water from river
7.35
4
Unknown sample
7.00
Table 2. Absorbance of Samples
Table 3. Calibration Curve Data*
Sample No.
Absorbance
Absorbance
3-
Concentration of PO4
Ions, ppm
(1 pt each)
1
1.33
1.0
0.050
2
3.26
2.0
0.093
0.89
3.0
0.137
4
0.099
4.0
0.185
5.0
0.230
*Provided by the instructor
II. Graph
Determination of Phosphate Concentration
3-
1. Using Excel, plot the concentration of PO4* (x-axis) vs. absorbance (refer to Table 3) The olot
must show the equation of the best-fit (or trend) line of the graph. Attach the graph below
arrow_forward
© Macmillan Learning
The spectroscopic data in the table is generated with five solutions of known concentration.
Concentration (M)
0.0163
m =
0.0326
0.0652
0.130
0.261
Absorbance
0.1233
0.2143
0.5231
0.8887
2.008
Use a spreadsheet program, such as Microsoft Excel, to graph the data points and determine the equation of the best-fit line.
What is the slope of the linear regression line formed by these points?
M-1
arrow_forward
Which of the following is incorrect:
General steps in qualitative analysis include: Method selection, sampling,
sample processing, calibration & measurement.
b. Quantitative analytical methods include: gravimetric, volumetric,
spectroscopic and electroanalytical methods.
c. The first step in sample processing is sample preparation.
d. Replication improves the quality of results and their reliability.
e. In quantitative analysis there should be a direct proportionality between a
measured property and the concentration or amount of substance.
Please fill in the spa ces one of the following characters (a or b or e or d or e)
arrow_forward
C030
BY NC SA
S. Alvanipour, Y, Gedeon, G. Zoorob, CC BY-NC-SA 4.0
B. Determination of unknown acid concentration
Unknown Acid number
Volume of acid used
(ml)
Initial buret reading
(mL)
Final buret reading
(ml)
Volume of NaOH used
(ml)
Molarity of NaOH
Trial 1
Unknown
Quid
25.0ml
Ome
12.4ml
12.4ml
-10m
Calculated molarity of
HCI (M)
Show a sample calculation of HCI molarity
AVERAGE MOLARITY OF Unknown HCI
Show a sample calculation of NaOH molarity:
Trial 2
Unknown
25-am
omo
12.5ml
12.5ml
-lom
Trial 3
Unknown
af
20% ome
come
12.630
12.6ml
-tom
arrow_forward
Line equation?
arrow_forward
Consider the spectra of a dye mixture shown below.
Dye 1
Dye 2
• Question 12
A. Complete the following table:
Dye
Analytical Wavelength (nm)
Absorbance
B. Calculate the concentration of Dye 1 in the mixture, if the Beer's Law plot is a straight line with
the follow equation:
Absorbance 1.457 x 104 * (Molarity) + 0.0099
M
C.If Dye 1 has the chemical formula of of C20HelaO,Naz (880 8/mol. calculate the number of
grams of dye 1 necessary to make 500.0 ml of this mixture.
Question Help: Message instructor
I Calculator
Submit Ouestion
arrow_forward
19. What type of sample analysis usually operates on the 10-4 g scale of sample size?
A. macro analysis
C. micro analysis
B. semi-micro
D. ultra-micro
analysis
analysis
20. Under what classification does a constituent group when its concentration is between
100 ppm and 1ppb?
A. Major constituents B. Interferences
C. Minor
D. Trace
constituents
constituent
arrow_forward
Please help me with letter C table
arrow_forward
Match the letter label to the value of / it stands for.
2
0
1
3
arrow_forward
I need accurate answer with full detail. This is my only chance to get it right.
arrow_forward
= 1
5
Absorbance vs. Concentration
y = 0.2755x
0.2-
0.1-
1.400
2.000
1.800
1.600
1.000
0.200
1.200
0.400
0.600
Concentration (M)
Using the calibration curve, calculate the molar concentration for a solution with a measured absorbance of 0.374. Round your answer to 3 significant digits
Concentration = M
X
Sub
Absorbance
0.7-
0.6-
0.5
0.4-
0.800
arrow_forward
Part B
The seventh ionization energy of manganese.
Express your answer as a chemical equation. Identify all of the phases in your answer.
ΑΣφ
OA chemical reaction does not occur for this question.
Submit
Request Answer
< Return to Assignment
Provide Feedback
Ма
Word
B.
amazon
U田
arrow_forward
Using the lab instructions and data table with data, please help me complete part 1 and 2 of my lab worksheet
arrow_forward
ne
te
1
A
kax
e 1 of 2
Insert Draw
a. Carbocal
!
AutoSave OFF
A
Font
b. (See section 10.4)
173 words
Design Layout >>
Paragraph
c. (See section 5.14)
Z
e
Focus
Styles
OH
Br₂
light
The partia
Dictate Sensitivity
PBr3
Tell me
=
Cheyy
independent experiment Sally wanted
Chapter Summary...
2-methylpe X
Comments
E
Editor
10
+
Q D
Share
100%
Show
arrow_forward
Case #5
Case #6
Jeepers! Someone snuck into
the Movie Madness studio and
Someone stole the net from the
High Wire act at the Crazy Circus!
Without it, the show can't go on!
stole the reels for the new film!
The CSI team found a liquid at
At this crime scene, there were
traces of a liquid, 9 drops (at ,059
drop) were found to contain
279C, 119790, and ,06039H, What
was the empirical formula of the
compound? Who committed the
heinous act?
this crime scene, When the
,77009 sample was analyzed, it
was found to contain ,04679H
and ,724390, What was the
empirical formula? Who should
the police question?
a
Case #7
Case #8
Old Man Higgins had his
favorite cane taken from him
when he was out for a walk
through Sinister Park, The CSI
team found traces of a uhite
powder at this crime scene, To
be 61,67%CL, A flame test
yielded a yellow flame,
indicating the remaining
portion was sodium, What was
the empirical formula of the
compound? What kind of
person would steal an antique
cane?
The original…
arrow_forward
What should the concentration be for the acid? Calculate your percent error. Show work.
arrow_forward
Please don't provide handwritten solution.....
Please draw stereographic projection of 4/m 3 2/m and m 3 m. Mark the intercepts and draw your conclusions. Compare and contrast both point symmetries.
arrow_forward
Using your determined value of Ksp = 3.7x10^-11 calculate how many milligrams of silver chromate will dissolve in 10.0mL of water (show work).
Please answer fast I give you upvote.
arrow_forward
Dangerous Paint Stripper Jessica has a summer job working for the
city parks program. She has been using a cleaner called “Graffiti Gone” to
remove graffiti from the bathrooms. She has to take a lot of breaks, because
the chemical makes her throat burn. It also makes her feel dizzy sometimes,
especially when the bathrooms don’t have very many windows. On the label, she
sees that the cleaner has methylene chloride in it. She feels like she’s
managing to get the work done, but she is worried about feeling dizzy. She
wants to find out more about this chemical, what harm it can cause, and
whether there are safer ways to do this work.
Questions for following story.
1. What went right in this situation?
2. What went wrong in this situation?
3. What steps should be taken in this workplace to make sure employees are
better protected and prepared the next time?
arrow_forward
SEE MORE QUESTIONS
Recommended textbooks for you
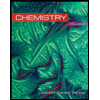
Chemistry
Chemistry
ISBN:9781305957404
Author:Steven S. Zumdahl, Susan A. Zumdahl, Donald J. DeCoste
Publisher:Cengage Learning
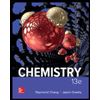
Chemistry
Chemistry
ISBN:9781259911156
Author:Raymond Chang Dr., Jason Overby Professor
Publisher:McGraw-Hill Education
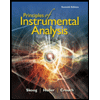
Principles of Instrumental Analysis
Chemistry
ISBN:9781305577213
Author:Douglas A. Skoog, F. James Holler, Stanley R. Crouch
Publisher:Cengage Learning
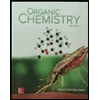
Organic Chemistry
Chemistry
ISBN:9780078021558
Author:Janice Gorzynski Smith Dr.
Publisher:McGraw-Hill Education
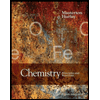
Chemistry: Principles and Reactions
Chemistry
ISBN:9781305079373
Author:William L. Masterton, Cecile N. Hurley
Publisher:Cengage Learning
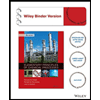
Elementary Principles of Chemical Processes, Bind...
Chemistry
ISBN:9781118431221
Author:Richard M. Felder, Ronald W. Rousseau, Lisa G. Bullard
Publisher:WILEY
Related Questions
- 7. Don believes that fewer than one-third of the students at his college hold part-time jobs while in school. To investigate this, he randomly surveyed 116 students and found that 32 students held part-time jobs. a. ( - Provide a 90% confidence interval for the population proportion of students that hold a part-time job. b. 1 (a). ;Give the correct interpretation of the interval in part c. Extra Credit: Does this interval support Don's claim? Why?arrow_forward20,18,2,4,15,15,10 Using these data, construct a 80% confidence interval for the average net change in a student's score after completing the course. Assume the population is approximately normal. Construct the 80% confidence interval. Round your answer to one decimal place.arrow_forwardHP TrueVision HD 4 Texas Workfor Mộc Homework × W. College Physic mical App AN Careers eagleonline.hccs.edu/courses/188795/assignments/3601808 valnabie diler reD 4 dl 10.1Tam Constants | Periodic Table Part O Complete the fourth row. Complete the following table by calculating the missing entries and indicating whether the solution is acidic or basic. Express your answer using two decimal places. Acidic or [H+] [OH-] pH pOH basic? 7.4x10-3 ΑΣφ 3.3x10-10 M pH 8.25 5.73 Is to fa ho II 10 insertarrow_forward
- 1. Data & Results 4.0 mglmL Concentration of stock solution (include units): Table 1-Beer's Law Plot Data V Volume of standard solution dispensed (mL) M, Diluted concentration (include units) Sample Absorbance A 2.00 mL 0.084 4.00 mL 0.173 l6.00 mL 0.259 8.00 mL 0.340 E 10.00 mL 0.418 Equation of trendline from Beer's Law plot: whats the diluted concentration and equation of trendline from the beers law plot once created?arrow_forwardi need help calculating the molar concentration for part Carrow_forwardI need help. Using a standard curve: Another student produced the standard curve shown here ( Fig.2.2) which shows the linear relationship between absorbance and Cu2+ concentration. They then measured the absorbance of their unknown solution to be 0.48126 AU. Use their trendline equation to calculate the concentration (M) of Cu + in their Unknown Solution. Show your work and consider sig figs.arrow_forward
- Determine the standard concentration of A through E and then the absorbance. I just need clarification on if I add together the two ml values given.arrow_forwardTable 1. pH of water samples Sample No. Water Samples pH 1 Tap water from household 6.59 2 Water from creek 7.35 3 Water from river 7.35 4 Unknown sample 7.00 Table 2. Absorbance of Samples Table 3. Calibration Curve Data* Sample No. Absorbance Absorbance 3- Concentration of PO4 Ions, ppm (1 pt each) 1 1.33 1.0 0.050 2 3.26 2.0 0.093 0.89 3.0 0.137 4 0.099 4.0 0.185 5.0 0.230 *Provided by the instructor II. Graph Determination of Phosphate Concentration 3- 1. Using Excel, plot the concentration of PO4* (x-axis) vs. absorbance (refer to Table 3) The olot must show the equation of the best-fit (or trend) line of the graph. Attach the graph belowarrow_forward© Macmillan Learning The spectroscopic data in the table is generated with five solutions of known concentration. Concentration (M) 0.0163 m = 0.0326 0.0652 0.130 0.261 Absorbance 0.1233 0.2143 0.5231 0.8887 2.008 Use a spreadsheet program, such as Microsoft Excel, to graph the data points and determine the equation of the best-fit line. What is the slope of the linear regression line formed by these points? M-1arrow_forward
- Which of the following is incorrect: General steps in qualitative analysis include: Method selection, sampling, sample processing, calibration & measurement. b. Quantitative analytical methods include: gravimetric, volumetric, spectroscopic and electroanalytical methods. c. The first step in sample processing is sample preparation. d. Replication improves the quality of results and their reliability. e. In quantitative analysis there should be a direct proportionality between a measured property and the concentration or amount of substance. Please fill in the spa ces one of the following characters (a or b or e or d or e)arrow_forwardC030 BY NC SA S. Alvanipour, Y, Gedeon, G. Zoorob, CC BY-NC-SA 4.0 B. Determination of unknown acid concentration Unknown Acid number Volume of acid used (ml) Initial buret reading (mL) Final buret reading (ml) Volume of NaOH used (ml) Molarity of NaOH Trial 1 Unknown Quid 25.0ml Ome 12.4ml 12.4ml -10m Calculated molarity of HCI (M) Show a sample calculation of HCI molarity AVERAGE MOLARITY OF Unknown HCI Show a sample calculation of NaOH molarity: Trial 2 Unknown 25-am omo 12.5ml 12.5ml -lom Trial 3 Unknown af 20% ome come 12.630 12.6ml -tomarrow_forwardLine equation?arrow_forward
arrow_back_ios
SEE MORE QUESTIONS
arrow_forward_ios
Recommended textbooks for you
- ChemistryChemistryISBN:9781305957404Author:Steven S. Zumdahl, Susan A. Zumdahl, Donald J. DeCostePublisher:Cengage LearningChemistryChemistryISBN:9781259911156Author:Raymond Chang Dr., Jason Overby ProfessorPublisher:McGraw-Hill EducationPrinciples of Instrumental AnalysisChemistryISBN:9781305577213Author:Douglas A. Skoog, F. James Holler, Stanley R. CrouchPublisher:Cengage Learning
- Organic ChemistryChemistryISBN:9780078021558Author:Janice Gorzynski Smith Dr.Publisher:McGraw-Hill EducationChemistry: Principles and ReactionsChemistryISBN:9781305079373Author:William L. Masterton, Cecile N. HurleyPublisher:Cengage LearningElementary Principles of Chemical Processes, Bind...ChemistryISBN:9781118431221Author:Richard M. Felder, Ronald W. Rousseau, Lisa G. BullardPublisher:WILEY
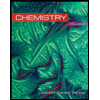
Chemistry
Chemistry
ISBN:9781305957404
Author:Steven S. Zumdahl, Susan A. Zumdahl, Donald J. DeCoste
Publisher:Cengage Learning
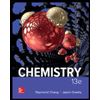
Chemistry
Chemistry
ISBN:9781259911156
Author:Raymond Chang Dr., Jason Overby Professor
Publisher:McGraw-Hill Education
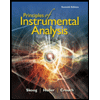
Principles of Instrumental Analysis
Chemistry
ISBN:9781305577213
Author:Douglas A. Skoog, F. James Holler, Stanley R. Crouch
Publisher:Cengage Learning
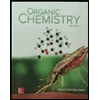
Organic Chemistry
Chemistry
ISBN:9780078021558
Author:Janice Gorzynski Smith Dr.
Publisher:McGraw-Hill Education
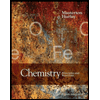
Chemistry: Principles and Reactions
Chemistry
ISBN:9781305079373
Author:William L. Masterton, Cecile N. Hurley
Publisher:Cengage Learning
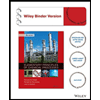
Elementary Principles of Chemical Processes, Bind...
Chemistry
ISBN:9781118431221
Author:Richard M. Felder, Ronald W. Rousseau, Lisa G. Bullard
Publisher:WILEY