chapter 8 _231002_083834
pdf
keyboard_arrow_up
School
University of Alabama *
*We aren’t endorsed by this school
Course
2333
Subject
Biology
Date
Nov 24, 2024
Type
Pages
24
Uploaded by MajorFlyMaster764
The biochemistry of survival Crayfish can escape rapidly from danger by tail flipping, provided that the cells in their tail muscles can generate adenosine triphos-
phate (ATP) at high enough rates. The contractile apparatus in each muscle cell requires ATP at a high rate to have energy for rapid, pow-
erful contractions.
A startled crayfish swims backward at jetlike speed by flipping its tail in a series of powerful contractions. In this way, a crayfish discovered under a rock in a stream can move to anoth-
er rock and disappear almost before its presence is appreciated. The tail muscles, which account for 25% of a crayfish’s weight in some species, are a powerful tool for escape and survival. To contract, however, the crayfish’s muscles—like all muscles—require adenosine triphosphate (ATP) as their immediate source of energy for contraction. When a crayfish is startled, its first tail flip occurs instantly and is followed in short order by multiple additional flips. Each of these massive contractions requires a substantial amount of ATP. How is the ATP made available so promptly? The same question can be asked about the ATP that a human sprinter requires to run 100 meters (m) in 10 seconds (s).
Burst exercise
is a general term that refers to sudden, intense exercise. Besides escaping crayfish and sprinting people, burst exercise is illustrated by salmon leaping waterfalls during their upstream journey, cheetahs racing toward antelopes, and scallops jetting away from danger by clapping their shells. In most animals, burst exercise cannot be continued for long periods. Crayfish escaping by flipping their tails become exhausted after 15–30 flips, just as human sprinters are exhausted or nearly exhausted at the ends of their brief races.
8
Aerobic and Anaerobic Forms of Metabolism 08_HILL4E.indd 189
4/1/16 1
A second general form of physical activity is sustained exercise
, defined to be exercise that can be continued at a steady rate for a long period. Jogging by humans, migratory flight by birds, and steady cruising by fish or crayfish are examples. During sustained exercise, the locomotory muscles must be supplied with ATP minute after minute for long, uninterrupted periods. What are the mechanisms that supply ATP in this way?
Comparing sustained exercise and burst exercise, do the mecha-
nisms that supply ATP during sustained exercise differ from the higher-intensity, shorter-duration mechanisms used during burst exercise? Do different types of animals differ in their ATP-producing mechanisms? Is exercise ever forced to stop because of limitations in ATP-generating mechanisms? These are some of the questions we discuss in this chapter.
ATP is not transported from one cell to another. Each cell, therefore, must make its own ATP
. This is one of the most critically important properties of ATP physiology. Another key property is that ATP is not stored by cells to any substantial extent
. Because of these two properties, the rate
at which a cell can do muscular work (or carry out other forms of ATP-requiring physiological work) at any given moment depends strictly on the rate at which that very cell
is able to produce ATP at the moment.
The following two complementary reactions, taken together, serve as a crucial energy shuttle
and energy transduction
mechanism in cells:
ADP + P
i
+ energy from foodstuff molecules →
ATP (8.1)
ATP →
ADP + P
i
+ energy usable by cell processes (8.2)
Each cell uses energy from carbohydrates, lipids, or other food-
stuff molecules to drive Equation 8.1, causing ATP to be formed from adenosine diphosphate (ADP) and inorganic phosphate ions (HPO
4
2–
), symbolized P
i
. In this way, the energy from the bonds of the foodstuff molecules is moved into bonds of ATP in the cell and becomes poised for use in physiological work. Energy-demanding processes in the cell—such as muscle contraction or ion pumping—
then split the ATP, as shown in Equation 8.2, thereby releasing the energy they need. Cellular energy-demanding processes are not able to draw energy directly from the bonds of foodstuff molecules. The energy-demanding processes, therefore, (1) are utterly dependent on the cellular mechanisms that drive Equation 8.1 and (2) can take place only as fast as those mechanisms supply ATP.
Mechanisms of ATP Production and Their Implications
There are two major categories of catabolic, biochemical pathways by which animal cells release energy from foodstuff molecules to synthesize ATP and thus make energy available for the perfor-
mance of physiological work. Some pathways, termed aerobic
, require O
2
; others, termed anaerobic
, can function without O
2
. In this section we examine the mechanistic features of the aerobic and anaerobic catabolic pathways. In later sections of this chapter we will look at the interplay between the aerobic and anaerobic modes of energy release when animals engage in burst or sus-
tained exercise, or when they face environmental stresses such as O
2
deficiency. There is a compelling reason to start with study of the mechanistic features of the pathways of ATP production: The overall performance of animals during exercise and in other situations often depends on the mechanistic peculiarities of the particular biochemical pathways by which energy is extracted for use from foodstuff molecules.
Aerobic catabolism consists of four major sets of reactions
Each cell in most animals possesses aerobic catabolic pathways
: pathways that, by use of O
2
, completely oxidize foodstuff molecules to CO
2
and H
2
O and capture in ATP bonds much of the chemi-
cal energy thereby released. These aerobic pathways typically can oxidize all
the major classes of foodstuffs. Here, for simplicity, we emphasize just the catabolism of carbohydrates. Moreover, our aim is to provide an overview, not to duplicate detailed treatments avail-
able in texts of cellular physiology or biochemistry. The principal aerobic catabolic pathway can be subdivided into four major sets of reactions: (1) glycolysis
, (2) the
Krebs cycle (
citric acid cycle
), (3) the electron-transport chain
, and (4) oxidative phosphorylation
.
GLYCOLYSIS Glycolysis
is the series of enzymatically catalyzed reactions shown in FIGURE 8.1
, in which glucose (or glycogen) is converted to pyruvic acid. The enzymes and reactions of glycolysis occur in the cytosol. The first step in glycolysis is that glucose is phosphorylated at the cost
of an ATP molecule to form glucose-
6-phosphate. Glucose-6-phosphate is then converted to fructose-
6-phosphate, and the latter is phosphorylated—also at the cost
of an ATP molecule—to form fructose-1,6-diphosphate. The latter is cleaved to form two three-carbon molecules: dihydroxyacetone phosphate and glyceraldehyde-3-phosphate. These compounds are interconvertible, and when glucose is being catabolized for release of energy, the former is converted to the latter, yielding two mol-
ecules of glyceraldehyde-3-phosphate. The reactions subsequent to glyceraldehyde-3-phosphate in Figure 8.1 are all multiplied by 2 to emphasize that two molecules follow these pathways for each glucose molecule catabolized.
The reaction that uses glyceraldehyde-3-phosphate is
the only oxidation reaction
in glycolysis and is particularly significant for understanding not only aerobic but also anaerobic catabolism. Each molecule of glyceraldehyde-3-phosphate is oxidized, with the addition of inorganic phosphate (P
i
), to a three-carbon di-
phosphate: 1,3-diphosphoglyceric acid. Although this reaction is an oxidation reaction, it does not itself require O
2
. Instead, it occurs by the simultaneous reduction of one molecule of nicotinamide adenine dinucleotide (NAD) per molecule of glyceraldehyde-3-phosphate. NAD—which a cell synthesizes from the vitamin niacin—is a relatively small, nonprotein mol-
ecule that undergoes reversible oxidation and reduction. When a molecule of glyceraldehyde-3-phosphate is oxidized, two hydrogen atoms are removed from it and transferred to NAD, reducing the NAD to form NADH
2
.
1
The two hydrogens remain bound to NADH
2
only temporarily because they are soon passed to another compound, regenerating NAD.
An alternative way to think about an oxidation–reduction reac-
tion—such as the oxidation of glyceraldehyde-3-phosphate—is that electrons are transferred. In the reaction under discussion, NAD 1
The reduction of NAD is symbolized “NAD + 2 H →
NADH
2
” or “NAD →
NADH
2
” in this book because these simplified expressions compactly emphasize the features of relevance for us. The true reaction is NAD
+
+ 2H →
NADH + H
+
.
08_HILL4E.indd 190
4/1/16 1
serves as the immediate electron acceptor
because it combines with electrons (hydrogens) removed from glyceraldehyde-3-phosphate. The 1,3-diphosphoglyceric acid formed from the oxidation of glyceraldehyde-3-phosphate is next converted to a monophosphate, 3-phosphoglyceric acid, with the formation of one ATP per mol-
ecule. The 3-phosphoglyceric acid is then converted in two steps to phosphoenolpyruvic acid, and the latter reacts to form pyruvic acid, again with the formation of one ATP per molecule.
Three important consequences of glycolysis deserve note:
Each molecule of glucose is converted into two molecules of pyruvic acid.
Two molecules of NAD are reduced to NADH
2
per molecule of glucose catabolized.
Two molecules of ATP are used and four are formed for each glucose processed, providing a net yield
of two ATP
molecules per glucose
molecule
.
THE KREBS CYCLE (CITRIC ACID CYCLE) During aerobic catabolism, the pyruvic acid formed by glycolysis enters the mitochondria by facilitated diffusion (mediated by a carrier protein just recently defined). The pyruvic acid is then oxidized in the mi-
tochondria by a cyclic series of enzymatically catalyzed reactions called the Krebs cycle
(
citric acid cycle
). This set of reactions, named after Hans Krebs, who in 1937 was the first to envision its features, is diagrammed in FIGURE 8.2
. For simplicity the reac-
tions involved in the oxidation of just one molecule of pyruvic acid are shown, although two molecules are in fact processed for each molecule of glucose.
This is the number of carbon atoms in each compound. 2
2
2
2
ATP
ADP
ATP
ADP
ATP
ADP
ATP
ADP
P
i
2
Glucose (6C)
Glucose-6-phosphate (6C)
Fructose-6-phosphate (6C)
Fructose-1,6-diphosphate (6C)
Glyceraldehyde-3-phosphate (3C)
Dihydroxy-
acetone phosphate (3C)
2 1,3-Diphosphoglyceric acid (3C)
2 NAD
2 NADH
2
2 3-Phosphoglyceric acid (3C)
2 2-Phosphoglyceric acid (3C)
2 H
2
O
2 Phosphoenolpyruvic acid (3C)
2 Pyruvic acid (3C)
Hexokinase
Phosphofructokinase
Glyceraldehyde-3-
phosphate dehydrogenase
Pyruvate kinase
Phosphoglycerate kinase
Enolase
FIGURE 8.1 The major reactions of glycolysis Each reac-
tion requires catalysis by an enzyme protein. The three-dimensional structures of six of these enzyme proteins are shown. Subunits of an enzyme are shown in different colors. The expression for reduction of NAD, NAD →
NADH
2
, is shorthand; the actual reaction is NAD
+
+ 2 H →
NADH + H
+
. P
i
= inorganic phosphate. (Protein structures from Erlandsen et al. 2000.)
GTP
GDP
*
Pyruvic acid (3C)
NAD
NAD
NAD
NADH
2
NAD
Coenzyme A
Coenzyme A
Acetyl coenzyme A (2C)
CO
2
Oxaloacetate (4C)
NADH
2
NADH
2
NADH
2
Coenzyme A
Coenzyme A
CO
2
CO
2
Succinyl coenzyme A (4C) Succinate (4C) FAD
FADH
2
Fumarate (4C)
Malate (4C)
Citrate (6C)
Isocitrate (6C)
α
-Ketoglutarate (5C)
*
*
FIGURE 8.2 The major reactions of the Krebs cycle (citric acid cycle) A molecule of H
2
O enters the reactions at each aster-
isk (*). The expression for reduction of NAD, NAD →
NADH
2
, is short-
hand; the actual reaction is NAD
+
+ 2 H →
NADH + H
+
.
08_HILL4E.indd 191
4/1/16 1
Your preview ends here
Eager to read complete document? Join bartleby learn and gain access to the full version
- Access to all documents
- Unlimited textbook solutions
- 24/7 expert homework help
Pyruvic acid enters the Krebs cycle by participating in a complex set of reactions in which it is oxidatively decarboxylated, forming CO
2
and a two-carbon acetyl group that is combined with coenzyme A in the form of acetyl coenzyme A.
2
In the process, a molecule of NAD is reduced. Acetyl coenzyme A then reacts with oxaloacetate, with the end result that coenzyme A is released and the acetyl group is condensed with oxaloacetate (four-carbon) to form citrate (six-carbon). In the ensuing series of reactions, oxaloacetate is ultimately regenerated and then again can combine with acetyl coenzyme A. For our purposes, there is no need to review the reactions stepwise. It is more important to emphasize the overall outcomes of the reactions:
The six carbons of each glucose molecule catabolized emerge in the form of six molecules of CO
2
as the pyruvic acid molecules produced by glycolysis are processed by the Krebs cycle. The CO
2
is formed by decarboxylation reactions. Such reactions occur at two points in the Krebs cycle: in the conversion of isocitrate to α
-ketoglutarate and in the conversion of α
-ketoglutarate to succinyl coenzyme A. These two decarboxylations, plus the one in the reaction of pyruvic acid to form acetyl coenzyme A, account for the formation of three molecules of CO
2
for every molecule of pyruvic acid processed (thus six molecules of CO
2
formed per glucose molecule).
For each glucose molecule catabolized, the Krebs cycle produces eight molecules of NADH
2
and two molecules of FADH
2
. Oxidation reactions occur at four points in the Krebs cycle. At three of these, NAD is reduced, forming NADH
2
. At one (the oxidation of succinate to fumarate), another small, nonprotein molecule that undergoes reversible oxidation and reduction—flavin adenine dinucleotide (FAD)—is reduced, forming FADH
2
.
3
Recall also that one NADH
2
is formed in the reaction of pyruvic acid to form acetyl coenzyme A. Considering all the oxidation reactions, the processing of each pyruvic acid molecule results in four NADH
2
and one FADH
2
(thus eight NADH
2
and two FADH
2
per glucose molecule).
Two molecules of ATP are produced in the Krebs cycle for each molecule of glucose catabolized
. Guanosine triphosphate (GTP) is formed from guanosine diphosphate (GDP) when succinyl coenzyme A reacts to form succinate in the Krebs cycle. GTP donates its terminal phosphate group to ADP, resulting in GDP and ATP. Thus one molecule of ATP is generated for each molecule of pyruvic acid processed (two ATPs per glucose).
ELECTRON TRANSPORT, OXIDATIVE PHOSPHORYLATION, AND THE ROLE OF O
2
The final two of the four sets of reactions in the aerobic catabolic pathway are the electron-transport chain
and oxidative phosphorylation
. We discuss them together because they are often tightly linked.
A paradox you may have noticed is that thus far, in discussing aerobic
catabolism, we have not mentioned the involvement of 2
Coenzyme A, an essential compound for aerobic metabolism, is synthesized from pantothenic acid, a B vitamin.
3
Flavin adenine dinucleotide is synthesized from riboflavin (vitamin B
2
).
O
2
. The reason is that O
2
is in fact not a participant in any of the reactions of glycolysis or the Krebs cycle; in a very narrow sense, all those reactions can proceed without O
2
. Nonetheless, O
2
is essential. The reason it is essential lies in the disposition of the reduced NADH
2
and FADH
2
molecules.
NADH
2
and FADH
2
cannot serve as final resting places for electrons because NAD and FAD are present in only limited quantities in a cell. Whenever one of the mainstream molecules in glycolysis or the Krebs cycle is oxidized, the electrons (or hydro-
gens) removed are transferred to NAD or FAD. As stressed earlier, therefore, NAD and FAD are the immediate
electron acceptors. However, if the NADH
2
and the FADH
2
thereby formed were simply allowed to accumulate, a cell would soon run out of NAD and FAD. Running out of NAD and FAD would bring glycolysis and the Krebs cycle to a halt because NAD and FAD are required. Thus NAD and FAD cannot serve as final
electron acceptors. The electron-transport chain regenerates NAD and FAD by removing electrons (hydrogens) from NADH
2
and FADH
2
. For the ordinary operation of the electron-transport chain, O
2
is required. In that way O
2
is necessary for glycolysis and the Krebs cycle to function as they do during aerobic catabolism.
Let’s now focus on the electron-transport chain
(
respiratory chain
) itself. It consists of a series of four major protein complexes (I–IV), plus other compounds, located in the inner membranes of mitochondria (
FIGURE 8.3
). A key property of the constituents of the electron-transport chain is that each is capable of undergoing reversible reduction and oxidation. The compounds function in a discrete order—a chain. The chain takes electrons from NADH
2
and FADH
2
and passes them in sequence from one compound to the next in a series of reductions and oxidations (see Figure 8.3). Finally, the last compound in the electron-transport chain, known as complex IV
or cytochrome oxidase
, passes the electrons—along with “accompanying” protons (H
+
ions)—to oxygen, reducing the O
2
to water.
4
In this way, O
2
acts as the
final electron acceptor
. The net effect of the operation of the electron-transport chain is to take electrons from NADH
2
and FADH
2
(thereby regenerating NAD and FAD) and pass the electrons to O
2
.
The special role played by O
2
is important. The constituents of the electron-transport chain (e.g., cytochrome b
-
c
1
and cytochrome oxidase), just like NAD and FAD, are present in limited quantities in a cell and therefore cannot act as terminal electron acceptors. In contrast, O
2
is continuously supplied to a cell, and the product of its reduction, water, can be voided into the environment, thereby carrying electrons out of the cell
. An adult person produces about 0.8 L of water per day in the process of voiding electrons from cells!
The electron-transport chain, in addition to reoxidizing NADH
2
and FADH
2
, is also pivotally involved in the transfer of energy
from the bonds of foodstuff molecules to ATP. Molecular O
2
has a much higher affinity for electrons than NAD or FAD. Accordingly, a large decline in free energy takes place as the electrons originally taken from foodstuff molecules are passed through the electron-
transport chain. Much of this energy is captured in bonds of ATP. The process of forming ATP from ADP by use of energy released 4
Two electrons and two protons combine with one oxygen atom
. A molecule of oxygen (O
2
), therefore, can react with four electrons and four protons.
08_HILL4E.indd 192
4/1/16 1
in the transport of electrons through the electron-transport chain is called oxidative phosphorylation
. How is the energy released in electron transport used by oxida-
tive phosphorylation? How, in other words, are the two processes coupled? According to the chemiosmotic hypothesis
currently favored by most biochemists, the coupling is achieved indirectly by a two-step process of the sort discussed in Box 5.1.
5
As energy is released in electron transport, it is used to pump protons across the inner mitochondrial membrane, creating—across that membrane—a proton electrochemical gradient that is far from equilibrium. This gradient represents an energy store (see Box 5.1). Driven by the electrochemical gradient, protons diffuse back across the membrane toward equilibrium by way of molecules of a membrane-spanning, ATP-synthesizing protein. In this process, energy from the proton 5
The term chemiosmotic hypothesis
refers to the entire process outlined in this paragraph. Box 5.1 is essential reading for fully understanding the hypothesis.
electrochemical gradient becomes energy of proton movement, and this proton motive energy is used by the ATP-synthesizing protein to synthesize ATP from ADP. Let’s now look at further details of electron transport and oxidative phosphorylation. Of the four protein complexes in the electron-transport chain, three (complexes I, III, and IV) use energy released from electron transport to pump protons across the inner mitochondrial membrane, as shown in Figure 8.3B. As already indicated, this proton pumping is away from equilibrium and builds
an electrochemical gradient, seen in FIGURE 8.4A
. Collectively, according to current estimates, the three complexes pump ten protons into the intermembrane space for each pair of electrons (or hydrogens) that flow the full length of the electron-
transport chain from NADH
2
to oxygen. Although protons in the intermembrane space (see Figure 8.4A) have a strong tendency to diffuse back across the inner mitochondrial membrane, they are largely unable to pass through the membrane itself. Their Complex I
(also called NADH-dehydrogenase or NADH-Q oxidoreductase) accepts hydrogens from NADH
2
, thereby regenerating NAD. The complex becomes reoxidized by passing hydrogens to ubiquinone. Complex IV
(also called cytochrome oxidase) passes electrons to elemental oxygen, forming water.
Complex II
(also called succinate dehydrogenase or succinic dehydrogenase) oxidizes succinate in the Krebs cycle, passing hydrogens to FAD to form FADH
2
, which then passes the hydrogens to ubiquinone. As electrons flow through Complexes I, III, and IV, they lose energy, which is used to pump protons (H
+
) from the mitochondrial matrix into the intermembrane space.
2 H
2e
–
2e
–
2e
–
2e
–
2 H
2 H
NADH
2
NAD
FAD
FADH
2
I
II
III
IV
Outer membrane
Intermembrane space
(B) Pumping of protons into the mitochondrial intermembrane space
Inner membrane
Matrix
(core)
Mitochondrion
Intermembrane space
Intermembrane space
Outer membrane
Inner membrane
Inner mitochondrial membrane
Matrix
Matrix
(core)
Succinate dehydrogenase
NADH- dehydrogenase
Cytochrome b-c
1
Ubiquinone
Cytochrome oxidase (cytochrome a-a
3
)
H
2
O
½
O
2
2 H
+
Cytochrome c
H
+
H
+
H
+
H
+
H
+
H
+
Cytoplasm
I
III
IV
FIGURE 8.3 The electron-transport chain As shown in (A), the electron-transport chain consists of four enzyme protein complexes, called complexes I through IV, embedded in the inner mitochondrial membrane, plus ubiquinone and cytochrome c
. The three purple complexes (I, III, and IV) capture energy from the flux of electrons to pump protons into the intermembrane space from the matrix across the inner membrane, as shown in (B), storing energy for synthesis of ATP. Ubiquinone, also called coenzyme Q, is a lipid-soluble compound that is mobile in the lipid core of the membrane. As also shown in (A), ubiquinone that is reduced by receiving hy-
drogens from complex I or FADH
2
becomes reoxidized by passing electrons to complex III, also called cytochrome b
-
c
1
. Complex III then passes the electrons to cytochrome c
(a wa-
ter-soluble compound), which in turn passes them to complex IV (also called cytochrome oxidase, cytochrome a
-
a
3
, COX, or cytochrome c
oxidase). e
–
= electron, H
+
= proton. (After Saraste 1999.)
08_HILL4E.indd 193
4/1/16 1
back-diffusion therefore occurs, as shown in FIGURE 8.4B
, via molecules of a membrane-spanning protein—
ATP synthase
—that is permeable to protons and that couples the motive energy of the diffusing protons to the synthesis of ATP from ADP.
6
A common mode of expressing the efficiency
of ATP production by oxidative phosphorylation is as a P/O ratio
(P = phosphate, O = oxygen), defined to be the number of ATP molecules formed per atom
of oxygen reduced to water. According to current estimates, in mammalian mitochondria, ATP synthase makes 1 ATP for every 4.3 protons that cross through it. As earlier noted, electron transport pumps 10 protons for each pair of electrons (or hydrogens) that pass through the electron-transport chain. Inasmuch as a pair of electrons (or hydrogens) combines with 1 atom of oxygen at the end of the chain, that’s 10 protons per O atom reduced. Those 10 protons, when they pass through ATP synthase, result in production of 2.3 ATP molecules (1 ATP per 4.3 protons). Thus the P/O ratio is 2.3. This value, P/O = 2.3, is currently considered to be the maximum P/O ratio for electrons that pass the entire length of the electron-transport chain from NADH
2
to oxygen.
Linkage of electron transport with oxidative phosphorylation is called coupling
. An important aspect of coupling is that it is capable of being graded
in its completeness (efficiency). Because of this graded nature, the P/O ratio can be lower than the maximum possible. In fact, a P/O ratio of 0 (zero) is observed at times in some 6
Spectacular simulations of the operation of this “splendid molecular machine” are available on the web.
types of cells, meaning that the P/O ratio can be as high as 2.3 or as low as 0. Electron transport and oxidative phosphorylation are said to be fully (“tightly”) coupled
when the P/O ratio is high and completely
uncoupled
when P/O = 0. Intermediate states of coupling are possible. Usually, coupling is relatively tight. The mitochondria of certain types of specialized
cells
express in their inner membrane—in addition to ATP synthase—a second type of membrane-spanning protein, uncoupling protein 1
(
UCP1
). This protein forms the basis for the most thoroughly understood mechanism of uncoupling. As shown in FIGURE 8.4C
, UCP1 provides a path for protons to diffuse from the intermembrane space across the inner membrane to the mitochondrial matrix without ATP synthesis.
7
Uncoupling opposes ATP production: In the fully uncoupled state (P/O = 0), even though electron transport continues to convert NADH
2
and FADH
2
to NAD and FAD—thereby per-
mitting continued oxidation of foodstuff molecules by glycolysis and the Krebs cycle—none of the energy released by electron transport is captured as ATP. The energy instead is immediately converted to heat. Uncoupling by UCP1 therefore is a specialized biochemical state that is ordinarily employed only when there is an advantage to converting food energy to heat rather than ATP. 7
There are at least four different hypotheses regarding how exactly UCP1 functions to achieve this outcome. The concept that UCP1 provides a straightforward back-diffusion path for protons is the simplest of the models.
Intermembrane space
Outer
mitochondrial membrane
Inner
mitochondrial membrane
Cytoplasm
Outer membrane
Intermembrane space
Inner membrane
Matrix
Matrix
(core)
Mitochondrion
Heat
Uncoupling protein (UCP1)
ATP
ATP
ADP
ATP synthase
ADP
(A)
The proton electrochemical gradient produced by electron transport: an energy store.
(B) Back-diffusion of protons via ATP synthase, providing energy for synthesis of ATP. This is the mechanism of oxidative phosphorylation.
(C)
Back-diffusion of protons in a specialized cell with UCP1, resulting in production of heat rather than ATP. This is the mechanism of uncoupling.
H
+
H
+
H
+
H
+
H
+
H
+
H
+
H
+
H
+
H
+
H
+
H
+
H
+
H
+
H
+
H
+
FIGURE 8.4 Principles of oxidative phosphorylation and uncoupling (A) Electron transport produces a proton electrochemical gradient, as shown in Figure 8.3, by pumping pro-
tons from the matrix into the intermembrane space. In (B) and (C), protons diffuse back to the matrix, driven by the proton electrochemical gradient, which is present in (B) and (C)—just as in (A)—but not shown for simplicity. Ordinarily, as seen in (B), protons diffuse back to the matrix through ATP synthase, which produces ATP by oxidative phosphorylation. In cells with uncou-
pling protein 1 (UCP1), as seen in (C), protons can also diffuse back to the matrix through UCP1, which produces no ATP and results in all the proton motive energy being dissipated as heat. 08_HILL4E.indd 194
4/1/16 1
Your preview ends here
Eager to read complete document? Join bartleby learn and gain access to the full version
- Access to all documents
- Unlimited textbook solutions
- 24/7 expert homework help
For example, uncoupling of oxidative phosphorylation is a major mechanism of increasing metabolic heat production in many mammals in cold seasons (see Chapter 10). It also sometimes serves as a mechanism of body-weight regulation by “burning off” the energy of excess food molecules.
8
In a tissue with UCP1, the degree of coupling is controlled by modulation of the extent to which protons back-diffuse by way of the two possible paths: ATP synthase and uncoupling protein (see Figure 8.4C). This control is mediated by a variety of agents, including fatty acids, hormones, and Ca
2+
ions.
Uncoupling by UCP1 is not the only mechanism that causes the P/O ratio to be less than maximum. A mechanism that is observed in a wider variety of cells than those with UCP1 is leakage
of protons across the inner mitochondrial membrane. In this case, protons diffuse across the membrane, from the intermembrane space to the matrix, by way of largely unknown, nonspecific pathways. Leakage reduces the amount of ATP synthesized (it reduces the P/O ratio) 8
Recall, for example, the rats mentioned in Chapter 7 that remain slim despite chronic overeating by undergoing diet-induced thermogenesis (DIT). Uncoupling is the principal mechanism of DIT in those animals.
because protons that leak do not pass through ATP synthase. In some tissues, 20% or more of the proton electrochemical gradient is dissipated by leakage, according to the evidence now available. This value for leakage is so high that it suggests there may be advantages achieved by allowing leakage to occur.
An important incidental effect of the processing of O
2
by mi-
tochondria is that highly reactive oxygen-containing compounds are produced as the processes we have described take place. These highly reactive entities—known as reactive oxygen species
(
ROS
)—
can react with and damage mitochondrial constituents (
BOX 8.1
). Moreover, some can exit the mitochondria and possibly react with and damage extramitochondrial cell constituents.
THE YIELD OF ATP FROM AEROBIC CATABOLISM Now let’s look at the total yield of ATP from the aerobic catabolism of glu-
cose when coupling is tight. For each molecule of glucose that is catabolized, 10 molecules of NADH
2
and 2 molecules of FADH
2
are formed in glycolysis and the Krebs cycle. When these mol-
ecules are processed via the electron-transport chain, the maxi-
mum yield of ATP by oxidative phosphorylation is 25 ATP mol-
Reactive Oxygen Species (ROS)
BOX 8.1
The ordinary metabolism of aerobic cells produces molecules—chemical spe-
cies—that because of the status of their oxygen atoms have a high potential to re-
act with (e.g., oxidize) cell lipids, proteins, and nucleic acids. These molecules are called reactive oxygen species
(
ROS
). ROS can have benign functions; they play signaling roles, for example. ROS are more famous, however, for their ability to dam-
age macromolecules on which health and life depend. ROS, in fact, are believed by some biologists to be the primary caus-
ative agents of aging. According to this hypothesis, we age because of incessant damage done to vital macromolecules by metabolically produced ROS; this dam-
age is repaired, but not entirely, causing defects to accumulate over time. ROS are also implicated in muscle fatigue and many other phenomena.
The superoxide radical (anion)—an O
2
molecule with an extra electron—is often the initial ROS produced by metabolism (see figure). Mitochondria routinely gener-
ate superoxide as a by-product of elec-
tron transport, and in turn, superoxide and its ROS products can injure mitochondria by reacting in destructive ways with mito-
chondrial macromolecules. Superoxide is produced also by several extramitochon-
drial reactions. Superoxide is converted to three other ROS: hydrogen peroxide, hydroxyl radicals, and peroxinitrite (see figure). All ROS are short-lived—sometimes exceedingly so—precisely because they are extraordinarily reactive. Hydroxyl radi-
cals, for example, exist for less than 1-bil-
lionth of a second after they are formed.
Cells possess antioxidant mecha-
nisms
—enzymatic and non-enzymatic mechanisms of detoxifying ROS. Enzyme antioxidants catalyze the transformation of ROS to less-reactive chemical forms. Su-
peroxide dismutase
, for example, converts superoxide to hydrogen peroxide, and catalase
converts hydrogen peroxide to water and O
2
(see figure). Non-enzymatic antioxidants often function in a sacrificial way: By reacting with ROS, they prevent the ROS from reacting with other, more critical molecules.
Oxidative stress
(
oxidant stress
) refers to damage inflicted by ROS on cel-
lular integrity. It occurs when the rate of ROS production exceeds the rate at which antioxidant mechanisms can dispose of ROS. Box Extension 8.1
discusses antioxi-
dants and the roles of ROS in more detail, including references.
An outline of the chemical relation-
ships of O
2
and four reactive oxygen species (ROS) The ROS are shown in boxes. A rough estimate of the average lifespan of each ROS is shown below the name of the ROS; for example, superoxide radicals typically react—and therefore cease to be superoxide radicals—within 1-millionth of a second (10
−6
s) after they are formed. Superoxide dismutase (SOD) and catalase are enzymes. A superscript dot next to a compound symbolizes an unpaired electron. The reactions are shown in outline only, not as fully balanced reac-
tions. (After Allen et al. 2008.)
O
2
O
2
+ H
2
O
Superoxide radical
(10
–6
s)
Hydroxyl radical
(10
–12
s)
Hydrogen peroxide
(10 s)
Peroxinitrite
(1 s)
O
2
•
–
ONOO
–
HO
•
NO
•
H
2
O
2
Superoxide
dismutase
Catalase
08_HILL4E.indd 195
4/1/16 1
ecules per glucose.
9
Even in tightly coupled mitochondria, this theoretical yield is probably not fully achieved in reality because of leakage. Nonetheless, for simplicity, we will use this value in this book without further qualification.
Besides the ATP molecules made by oxidative phosphorylation, additional ATP molecules are generated by phosphorylation reactions associated with several steps in glycolysis and the Krebs cycle: the reaction of 1,3-diphosphoglyceric acid to form 3-phosphoglyceric acid (see Figure 8.1), that of phosphoenolpyruvic acid to form py-
ruvic acid (see Figure 8.1), and that of succinyl coenzyme A to form succinate (see Figure 8.2). These phosphorylations, in contrast to oxidative phosphorylations, are known collectively as substrate-
level phosphorylations
because they occur immediately in the reactions of substrates of glycolysis and the Krebs cycle. Altogether, 6 ATP molecules are formed by substrate-level phosphorylation per molecule of glucose under aerobic conditions. In total, therefore, 31 molecules of ATP are generated for each glucose molecule catabolized. However, 2 ATP molecules are consumed at the start of glycolysis (see Figure 8.1). The net
yield of ATP by aerobic catabolism is therefore 29 molecules of ATP per glucose. The energetic efficiency
of this ATP production—
defined by Equation 7.1—is not altogether certain and can vary even in a tightly coupled cell, depending on metabolite concentrations and other conditions.
10
In glucose catabolism, assuming tight coupling, the efficiency is usually considered to be 60%–70%. That is, 60%–70% of the chemical energy released by oxidizing glucose to CO
2
and H
2
O is captured as chemical-bond energy of ATP.
O
2
deficiency poses two biochemical challenges: Impaired ATP synthesis and potential redox imbalance
What are the biochemical implications if cells are denied O
2
or sup-
plied with O
2
at an inadequate rate? To explore this question, let’s for simplicity consider cells deprived entirely of O
2
, even though for many animals this would be an unrealistically extreme state.
Without O
2
, electrons entering the electron-transport chain cannot be discharged at the end by the reduction of O
2
. Thus the electron-
transport chain becomes a dead end
for electrons. The consequences for most animals are easily summarized. Soon after the supply of O
2
is cut off, all the cytochromes (see Figure 8.3A) and other constituents of the electron-transport chain become fully reduced because electrons entering the chain accumulate. The electron-transport chain then can no longer accept further electrons. Two major consequences follow. First, oxidative phosphorylation cannot take place, eliminat-
9
This value is calculated using the latest estimates of P/O ratios: P/O = 2.3 for most NADH
2
oxidation, P/O = 1.4 for FADH
2
oxidation. The paper by Martin Brand in the References provides a full, accessible explanation. By way of background, note that although both NADH
2
and FADH
2
are oxidized by the electron-transport chain, their electrons enter the chain at different points, giving the compounds different P/O ratios; also, a factor discussed in biochemistry texts is that NADH
2
produced in glycolysis has a lower effective P/O ratio than most NADH
2
because the former is produced outside the mitochondria and its electrons must be shuttled in. The P/O ratios used here are lower than those traditionally used (P/O = 3 for most NADH
2
oxidation, P/O = 2 for FADH
2
oxidation) because of modern revisions in the estimates.
10
For example, ATP does not have a fixed energy value, as often implied by introductory biology texts. Instead, the “energy content” of ATP depends on how close, or far away, the ATP–ADP interconversion reaction is to chemical equilibrium, which in turn depends on relative concentrations of the reactants in a particular cell.
ing a cell’s ability to produce 25 of the 29 ATP molecules that can be produced in net fashion by the aerobic catabolism of glucose. Second, the electron-transport chain can no longer serve as a mechanism for reoxidizing the reduced molecules of NADH
2
and FADH
2
that are produced by glycolysis and the Krebs cycle. The cell’s supply of NAD and FAD is thus threatened. A complete failure to regenerate NAD and FAD would mean that a cell could produce no ATP at all, because NAD is needed for glycolysis, and both NAD and FAD are required for operation of the Krebs cycle.
The inability of the electron-transport chain to regenerate NAD and FAD from NADH
2
and FADH
2
in the absence of O
2
can be described as a problem of redox balance
, meaning reduction–oxidation balance
. Redox balance
is a key concept in the study of all cellular compounds that undergo alternating reduction and oxidation. By definition, a cell is in redox balance for such a compound if the cell possesses the means to remove electrons from the compound as fast as electrons are added to it.
Applying the concept specifically to NAD, a cell is in redox bal-
ance for NAD if it can convert NADH
2
back to NAD (a process that removes electrons) as fast as NAD is being converted to NADH
2
(a process that adds electrons). During aerobic catabolism, O
2
and the electron-transport chain together provide the means for maintaining redox balance for NAD and FAD, thereby ensuring that NAD and FAD are steadily available to perform their essential roles in glycolysis and the Krebs cycle. To make ATP without O
2
, a cell must possess alternative mechanisms that will permit redox balance to be maintained while at least some ATP-generating reactions continue to take place.
Certain tissues possess anaerobic catabolic pathways that synthesize ATP
Only some tissues have evolved an ability to make ATP at signifi-
cant rates without O
2
. The mammalian brain is a prime example of a tissue that lacks this ability. The brain succumbs rapidly when denied O
2
(as during cardiac arrest) because when O
2
is unavailable, the brain is unable to synthesize ATP at the rate it needs it. Many other vertebrate tissues possess an anaerobic catabolic pathway that can produce ATP at a substantial rate without O
2
. These other tissues are therefore less rigidly dependent on an O
2
supply than the mammalian brain is.
Anaerobic glycolysis is the principal anaerobic catabolic pathway of vertebrates
Vertebrate skeletal muscle and many other vertebrate tissues are able to use the substrate-level phosphorylations of glycolysis to transfer energy from the bonds of glucose to ATP at substan-
tial rates in the absence of O
2
. For a tissue to have this ability, it must have a way of maintaining redox balance for NAD without O
2
. Glycolysis requires NAD to serve as the immediate electron acceptor for the oxidation of glyceraldehyde-3-phosphate, and in using NAD in this way, it converts the NAD to NADH
2
(see Figure 8.1). Under anaerobic conditions in skeletal muscles and other such tissues, the NADH
2
molecules are reoxidized to NAD by passing their electrons (hydrogens) to pyruvic acid, reducing the latter to lactic acid (
FIGURE 8.5
). Pyruvic acid, in essence, is used as the final electron acceptor by the anaerobic cell. The entire sequence of reactions that converts glucose to lactic acid is called anaerobic glycolysis
.
08_HILL4E.indd 196
4/1/16 1
ATP
ADP
2
2
ATP
ADP
4
4
2 NAD
2 NAD
2 NADH
2
2 NADH
2
O
C
COOH
H
3
C
H
3
C
OH
C
H
COOH
2 Glyceraldehyde-3-phosphate
2 1,3-Diphosphoglyceric acid
2 Pyruvic acid
Lactate dehydrogenase
2 Lactic acid
FIGURE 8.5 Anaerobic glycolysis The reduction of pyruvic acid is catalyzed by the enzyme lactate dehydrogenase (LDH) and permits redox balance to be maintained without O
2
. The net yield of ATP is two ATP molecules per glucose molecule if the initial fuel is glucose itself, as shown here. If glycogen is used as fuel, there is a net yield of three ATPs per glucose unit catabolized.
Carbon chains from lactic acid may be used to rebuild stores of carbohydrates…
…or they may be oxidized.
ATP
ATP
Glucose or glycogen
Gluconeogenesis
Glycolysis
Pyruvic acid
Lactic acid
Oxidation by Krebs cycle and electron transport
CO
2
and H
2
O
FIGURE 8.6 Major paths by which lactic acid is metabolized when O
2
is available The green arrows show the paths followed by the carbon chains of lactic acid. The carbon chains are first converted to pyruvic acid—and then can be converted to glucose or oxidized.
The principal determinant of whether a cell can carry out anaerobic glycolysis at a substantial rate is its expression of the enzyme lactate dehydrogenase (LDH), the enzyme that catalyzes reduction of pyruvic acid. LDH occurs in multiple molecular forms, as discussed in detail in Chapter 2 (see page 50). For a cell to carry out anaerobic glycolysis, it must synthesize significant amounts of one or more LDH forms that are catalytically effective in converting pyruvic acid to lactic acid under the intracellular conditions that prevail during O
2
deprivation. In cells that meet these criteria, a true redox bal-
ance exists during anaerobic glycolysis. As Figure 8.5 reveals, one molecule of pyruvic acid is produced for each molecule of NAD that is reduced to NADH
2
during glycolysis. Thus the supply of pyruvic acid keeps exact pace with the need for it as an electron acceptor. A cell with appropriate amounts and types of LDH is thus able to convert every NADH
2
that it forms back to NAD without O
2
. This means that from a strictly biochemical perspective, the cell can maintain a steady production of ATP without O
2
.
What fuels can anaerobic glycolysis use, and how much ATP can it make? Glucose and glycogen are the only foodstuff molecules that can serve as fuels for anaerobic glycolysis under most condi-
tions. The amount of ATP synthesized in net fashion by anaerobic glycolysis is two molecules of ATP per glucose molecule catabolized (see Figure 8.5). This yield of ATP is vastly lower than that from the aerobic catabolism of glucose. However, as we will see, the anaerobic pathway can nonetheless be an impressively important source of ATP. The major reason for the comparatively low yield of ATP per glucose molecule during anaerobic glycolysis is that only a small fraction (about 7%) of the free energy available from glucose is released by the conversion of glucose to lactic acid. Lactic acid is itself an energy-rich molecule.
Anaerobic glycolysis is not the only mechanism of anaerobic catabolism in animals. However, we will take up other anaerobic pathways later and now address the important matter of the dis-
position of end products.
What happens to catabolic end products?
For an animal to make use of any ATP-producing pathway, it must possess satisfactory means of disposing of the chemical end products generated. The principal products of aerobic
catabolism, CO
2
and H
2
O, are fully oxidized and not capable of being tapped for further energy. Animals typically dispose of CO
2
and H
2
O by voiding them into the environment, as when we exhale CO
2
by breathing.
11
The end products of anaerobic catabolic pathways, in contrast to CO
2
and H
2
O, are always organic compounds that are far from fully oxidized and possess considerable further potential to yield energy. The high energy value of these products places a premium on retaining them in the body for future use as energy sources; excreting lactic acid, for instance, would be equivalent to voiding more than 90% of the energy value of catabolized carbohydrates. However, unlimited retention of the organic end products of anaerobic catabolism is usually not possible, because the end products typically exert harmful effects if allowed to accumulate to high concentrations. These two competing considerations seem to have been major operative factors in the evolution of animal strategies for disposing of anaerobic end products. A third factor of importance is time. An animal that makes only short-term use of anaerobic catabolic pathways is more likely to be able to retain all the end-product molecules without adverse effects than an animal that uses anaerobic catabolism for days or weeks on end.
THE DISPOSITION OF LACTIC ACID IN VERTEBRATES When vertebrates use anaerobic glycolysis, they universally retain the lactic acid they produce in their bodies, and they ultimately get rid of the lactic acid metabolically
. The metabolism of lactic acid requires O
2
and thus can take place only when and where O
2
becomes available. Lactic acid itself is a metabolic cul-de-sac (
FIGURE 8.6
), meaning that for lactic acid to be metabolized, it must first be converted back to pyruvic acid by a reversal of the very reaction that formed it. The 11
In the study of water balance, the H
2
O produced by aerobic catabolism is known as metabolic water or oxidation water
. This water is sometimes an important part of the water budget (see Chapter 27).
08_HILL4E.indd 197
4/1/16 1
Your preview ends here
Eager to read complete document? Join bartleby learn and gain access to the full version
- Access to all documents
- Unlimited textbook solutions
- 24/7 expert homework help
conversion of lactic acid to pyruvic acid is an oxidation reaction, with NAD acting as the immediate electron acceptor. After conversion to pyruvic acid, the carbon chains of lactic acid are metabolized by one of two major paths, both of which require O
2
(see Figure 8.6):
1. One path is for the carbon chains to be used to form glucose or glycogen, thereby replenishing a cell’s carbohydrate stores. The conversion of lactic acid or pyruvic acid to glucose or glycogen is a form of gluconeogenesis
(“new formation of glucose”). The process uses ATP
(six ATP molecules per glucose molecule formed). It requires O
2
because the ATP must be made aerobically.
2. The second path is for the carbon chains to be fully oxidized by way of the Krebs cycle and electron-transport chain. This process makes ATP
(27 ATP molecules per pair of lactic acid molecules). It requires O
2
because the electron-transport chain must be functional.
To determine the relative importance of the two paths followed by lactic acid, investigators inject animals with lactic acid containing the unusual carbon isotope 14
C. They then monitor the fractions of the 14
C that show up in CO
2
and carbohydrate. These fractions vary, even within single species, in ways that are still poorly understood. Lactic acid may be metabolized by the same tissues that produce it, or it may be carried by the blood to other tissues that metabolize it. Quite often, tissues that are well supplied with O
2
metabolize lactic acid made by other tissues that are deprived of O
2
; this can occur even while lactic acid production continues.
The functional roles of ATP-producing mechanisms depend on whether they operate in steady state or nonsteady state
One of the most consequential properties of any ATP-producing mechanism is whether or not it can operate in steady state
. Ide-
ally, a mechanism is in steady state if (1) it produces ATP as fast as ATP is used, (2) it uses raw materials (e.g., foodstuff molecules) no faster than they are replenished, (3) its chemical by-products (be-
sides ATP) are voided (or metabolically destroyed) as fast as they are made, and (4) it does not cause other changes in cell function that progress to the point of disrupting cell function. When a cell uses a steady-state mechanism
of ATP production, even as ATP is made and used in the cell, the cell remains essentially constant in its levels of ATP and of the precursors and by-products of ATP production, and it also remains essentially homeostatic in other ways as well. At least in principle, therefore, a steady-state mechanism can go on and on indefinitely, free of intrinsic limitations
.
In contrast, a nonsteady-state mechanism
of ATP produc-
tion depletes supplies, accumulates products, or otherwise alters the conditions of its own operation at rates fast enough that the mechanism is self
-limiting or self
-terminating. For this reason, a nonsteady-state mechanism cannot persist for long by comparison with steady-state ones.
The concepts of steady-state and nonsteady-state mechanisms are relative rather than absolute. Nonetheless, they provide useful organizing principles in the study of ATP synthesis. In vertebrates and many invertebrates, aerobic ATP production is usually a steady-state process, whereas anaerobic ATP production is usually a nonsteady-state process.
EXAMPLES FROM HUMAN BIOLOGY To understand the practical meaning of steady-state and nonsteady-state mechanisms better, let’s examine some examples in our own lives. Routine daily living provides a good illustration of a circumstance in which ATP is pro-
duced by aerobic catabolism functioning in steady state. Consider what you are doing now, for instance. As you read this page, unless you have bizarre reading habits, you are in an aerobic steady state. You are making all your ATP by aerobic catabolism; your needs for ATP are fully met; you are taking in O
2
at the rate you use it; you are voiding CO
2
and H
2
O approximately as you produce them; and your cells are not undergoing any progressive alterations that might dis-
rupt their function. The one way in which you may not be in steady state is in regard to the foodstuff molecules you are using as fuels. If a person is not eating while reading, fuel levels are obviously falling. However, the rate of decline during reading is very low relative to fuel stores, and intakes of fuels probably closely match uses when averaged over a day (including meals). Thus, even with respect to fuels, the departure from steady state is merely technical.
The capability of the aerobic catabolic pathways to operate in steady state is of great significance when we consider the ATP requirements of daily living. Because aerobic catabolism can sup-
ply ATP at the required rates while operating in steady state, it can go on and on without self-limitation and meet our ATP needs for a lifetime!
A mile race is a good example of an activity that is fueled sub-
stantially by anaerobic ATP production functioning in nonsteady state. As we will see in detail shortly, the rate at which ATP is used in a competitive mile race is far greater than the maximum rate at which aerobic catabolism can make ATP. Thus additional ATP must be provided by anaerobic glycolysis throughout such a race. Anaerobic glycolysis is a nonsteady-state mechanism of ATP synthesis during a mile race because its use progressively induces alterations in the cells of the working muscles—alterations that will ultimately render continued running physiologically impossible
.
12
The process is self-limiting. The exact alterations that are mechanisti-
cally responsible for this self-limitation have yet to be nailed down. As we will discuss later in greater detail, the alterations include (1) accumulation of lactic acid (probably not responsible for fatigue), (2) acidification (probably partly responsible for fatigue but not the major player), and (3) other changes that are probably the principal causative agents.
For now, two principal points regarding the mile race are im-
portant to see. First, the mile race contrasts with routine daily living in that it cannot go on indefinitely. Second, the key reason for this difference lies in the mechanisms of ATP production: nonsteady-
state during the mile race, steady-state during daily living.
Phosphagens provide an additional mechanism of ATP production without O
2
Phosphagens
are compounds that serve as temporary stores of high-energy phosphate bonds. They occur in the skeletal muscles of vertebrates and the muscles of many invertebrates. Creatine phos-
phate
(
FIGURE 8.7A
) is the phosphagen of vertebrate muscle and also occurs in some groups of invertebrates. The most widespread phosphagen of invertebrates (including the crayfish with which 12
Mile runners collapse near the finish line fairly often in championship races.
08_HILL4E.indd 198
4/1/16 1
westarted this chapter) is arginine phosphate
(see Figure 8.7A). Other phosphagens are known, especially among annelids. Phosphagens are synthesized by use of high-energy phosphate bonds taken from ATP, and later they can donate the phosphate bonds to ADP to form ATP. The reversible reactions for creatine phosphate and arginine phosphate, catalyzed by phosphagen kinases
(
creatine kinase
and arginine kinase
), are shown in FIGURE 8.7B
.
Chemical mass action determines the direction of a phosphagen reaction.
13
For example, consider the reaction involving creatine phosphate and creatine in Figure 8.7B. During times when a vertebrate muscle cell is at rest, when ATP supplies in the cell are relatively untaxed and ATP concentrations are high, the reaction is shifted to the left: ATP reacts with molecules of creatine, and consequently most of the creatine in the resting cell becomes phosphorylated. The molar concentration of creatine phosphate in a vertebrate muscle cell might then be three to six times the concentration of ATP. Later, during times when ATP supplies are taxed and ATP concentrations fall, the reaction is shifted to the right, forming ATP
without a simultaneous need for O
2
. Each ADP molecule in a vertebrate muscle cell can be rephosphorylated three to six times by this mechanism, assuming the concentration ratios we just mentioned. Anaerobic production of ATP in this way is a nonsteady-state mechanism because it exhausts the supply of creatine phosphate and causes buildup of the end product creatine.
Internal O
2
stores may be used to make ATP
For certain purposes, it is useful to recognize that aerobic catabolism can function in two different modes, steady-state and nonsteady-
state, depending on its source of O
2
. Up to now, whenever we have 13
See Chapter 2 (page 50) for a discussion of the meaning of mass action.
discussed aerobic catabolism, we have assumed that O
2
is taken into the body from the environment and distributed to cells as it is used. Aerobic catabolism is a steady-state mechanism under such conditions. Aerobic catabolism, however, can also draw its O
2
from preexisting stores of O
2
in a cell or tissue. Aerobic catabolism using O
2
stores is a nonsteady-state process because it depletes the O
2
stores on which it depends. Muscle cells often have enhanced O
2
stores because they contain myoglobin
, a red O
2
-binding pigment that is actually a type of hemoglobin (see Chapter 24). The myoglo-
bin binds with O
2
when O
2
is abundant. Aerobic catabolism then can draw on these O
2
stores at other times.
SUMMARY
Mechanisms of ATP Production and Their Implications
Aerobic catabolism occurs in four steps: (1) glycolysis, (2) the Krebs cycle, (3) electron transport, and (4) oxidative phosphorylation. It can oxidize carbohydrates, lipids, or proteins to produce ATP. The maximum net yield of ATP in carbohydrate oxidation is currently estimated to be 29 ATP molecules per glucose molecule.
Anaerobic glycolysis is a redox-balanced process by which ATP can be made without O
2
in certain tissues. Whether a tissue can employ anaerobic glycolysis depends on the amount and type of its lactate dehydrogenase (LDH). Anaerobic glycolysis typically can use only carbohydrate fuel. It releases only about 7% of the energy of glucose. Thus it produces just two ATP molecules per glucose molecule, and the lactic acid produced is itself an energy-rich compound.
Vertebrates retain the lactic acid that they produce. The metabolism of lactic acid requires O
2
, either to oxidize the lactic acid via the Krebs cycle—thereby producing ATP—or to convert lactic acid to glycogen or glucose (gluconeogenesis)—a process that uses ATP.
Phosphagens in muscle cells serve as temporary stores of high-energy phosphate bonds, which they can transfer to ADP to make ATP anaerobically.
Comparative Properties of Mechanisms of ATP Production
We have now identified four mechanisms of ATP production: (1) aerobic catabolism by use of O
2
acquired simultaneously from the environment, (2) anaerobic catabolism, (3) anaerobic ATP produc-
tion by use of phosphagen, and (4) aerobic ATP production by use of internal O
2
stores. To compare the functional properties of these four, we now discuss several key questions. TABLE 8.1
can be used as a summary of this discussion.
Question 1: What is each mechanism’s total possible ATP yield per episode of use?
If an animal uses a particular mechanism to make ATP and continues using the mechanism until no more ATP can be made, what is the total amount of ATP that can be produced? Because aerobic catabo-
lism using environmental O
2
operates in steady state, it is capable of supplying an indefinite amount of ATP (a lifetime’s amount). (B)
Creatine phosphate + ADP
Arginine phosphate + ADP
creatine + ATP
arginine + ATP
Arginine kinase
Creatine kinase
C
N
H
N
C
COOH
CH
3
NH
2
HN
C
N
H
C
H
COOH
HN
N
H
H
2
C
H
2
C
H
2
C
H
2
(A)
Creatine phosphate
Arginine phosphate
P
i
P
i
FIGURE 8.7 Two important phosphagens and the reactions they undergo (A) Creatine phosphate and arginine phosphate. (P
i = inorganic phosphate). (B) The reversible reactions by which these phosphagens are formed from ATP or can donate their phos-
phate groups to ADP to make ATP. The reactions are catalyzed by creatine kinase and arginine kinase.
08_HILL4E.indd 199
4/1/16 1
In contrast, the three mechanisms that operate in nonsteady state are limited in the amount of ATP they can produce during any one episode of use because of their mechanistic self-limitations. For example, the amount of ATP that can be made from phospha-
gen is limited by the quantity of phosphagen available; after the phosphagen originally present has been used, no more ATP can be made until (at some later time) the phosphagen is regenerated. Table 8.1 shows representative yields of ATP in humans. Phospha-
gen and O
2
stores permit only relatively small quantities of ATP to be made per episode of use. The yield from anaerobic glycolysis is larger but modest.
Question 2: How rapidly can ATP production be accelerated?
In vertebrates and many types of invertebrates (although not insects), aerobic catabolism using environmental O
2
requires a relatively long time (minutes) to accelerate its rate of ATP production fully to a new high level. Acceleration is slow because this mechanism of ATP production is not self-contained in cells
. The mechanism requires inputs of O
2
, and the processes of breathing and blood circulation—which supply the needed O
2
by transporting it from the environment to the cells—increase their rate of O
2
delivery gradually, not instantly
.
By contrast, the other three mechanisms of ATP production can accelerate very rapidly because all are self-contained in the cells
. At the start of a bout of exercise, for example, a vertebrate muscle cell contains not only all the enzymes of anaerobic glycolysis but also the glycogen fuel required. Nothing needs to be brought to the cell for anaerobic glycolysis to take place, and in fact a cell is capable of nearly instantly stepping up its glycolytic rate of ATP production to a high level. ATP production by use of phosphagen and O
2
stores can also accelerate very rapidly because the phosphagen and O
2
stores are already present in cells when the mechanisms are called into play.
In both vertebrates and crustaceans such as crayfish, the first stages of burst exercise receive their ATP supply principally from anaerobic glycolysis, phosphagen use (
BOX 8.2
), and use of O
2
stores. This is true because these are the three mechanisms of ATP production that can accelerate their rates of ATP production very rapidly. Vertebrates would be incapable
of burst exercise without these mechanisms. As bizarre as it might be to imagine, if aerobic catabolism using O
2
from the environment were the only means of making ATP, vertebrates would be unable to start running suddenly at top speed; instead, they would have to accelerate their running gradually over a period of minutes!
Question 3: What is each mechanism’s peak rate of ATP production (peak power)?
After a mechanism has accelerated its ATP production to the fastest rate possible, how fast can ATP be made? As shown in Table 8.1, the peak rate at which anaerobic glycolysis can make ATP is much greater than the peak rate of ATP production by aerobic catabolism using environmental O
2
, and the rate of ATP production by use of phosphagen is greater yet. Although the phosphagen mechanism cannot make a lot of ATP, it can make its contribution very rap-
idly; thus it can briefly support very intense exertion. Anaerobic glycolysis can make a modest amount of ATP at a high rate, and aerobic catabolism using environmental O
2
can make an indefinite amount at a relatively low rate.
Question 4: How rapidly can each mechanism be reinitialized?
Whenever ATP has been made by a nonsteady-state mechanism, cells are left in an altered state and must be returned to their origi-
nal state before the mechanism can be used again to full effect. When internal O
2
stores have been used, the stores must later be recharged. When phosphagen has been used, it must be remade. When lactic acid has accumulated, it must be metabolized. In a word, the nonsteady-state mechanisms must be reinitialized
.
The reinitialization of anaerobic glycolysis requires much more time than the reinitialization of the phosphagen or O
2
-store mechanisms. This is true because the length of time required to rid tissues of lactic acid is much greater than the time required to rebuild phosphagen or O
2
stores. In people, for example, a substantial accumulation of lactic acid may require 15–20 min for TABLE 8.1 Comparative properties of mechanisms of ATP production in vertebrates, including numerical estimates for some of the properties in human beings
Mechanism of ATP production
Mode of operation (mandatory or assumed)
Total possible ATP yield per episode of use
a
(moles)
Rate of acceleration of ATP production at onset of use
Peak rate of ATP production
b
(μmol ATP/g
•
min)
Rate of return to full potential for ATP production after use
Aerobic catabolism using O
2
from environment
Steady state
Very large (~200 in a marathon, > 4 ×
10
6
in a lifetime)
Slow
Moderate (30 with glycogen fuel, 20 with fatty acid fuel)
—
Anaerobic glycolysis
Nonsteady state
Moderate (1.5)
Fast
High (60)
Slow
Phosphagen use
Nonsteady state
Small (0.4)
Fast
Very high (96–360)
Fast
Aerobic catabolism using O
2
preexisting in body
Nonsteady state
Small (0.2)
Fast
High
Fast
a
Numerical estimates of total yields are computed from information in Åstrand and Rodahl 1986; a 75-kg person living 70 years is assumed.
b
Peak rates of production are from Hochachka and Somero 2002.
08_HILL4E.indd 200
4/1/16 1
Your preview ends here
Eager to read complete document? Join bartleby learn and gain access to the full version
- Access to all documents
- Unlimited textbook solutions
- 24/7 expert homework help
half dissipation and even 1–2 h for full dissipation, whereas the half-time for reconstituting phosphagen and O
2
stores is just 30 s. Fish, frogs, lizards, and other poikilothermic vertebrates require even more time than mammals to dissipate lactic acid; 1–10 h may be required for half dissipation.
Conclusion: All mechanisms have pros and cons
A review of Table 8.1 and the preceding discussion reveals that each mode of ATP production has pros and cons; none is superior in all respects. Let’s consider, for example, the relative pros and cons of the two major sources of ATP in vertebrates: aerobic catabolism using environmental O
2
and anaerobic glycolysis.
When one looks simply at biochemical maps, aerobic catabo-
lism tends to seem far superior to anaerobic glycolysis. Aerobic catabolism fully releases the energy of food molecules (producing 29 ATPs per glucose), whereas anaerobic glycolysis unlocks only a small fraction of the energy value of food molecules (producing 2 ATPs per glucose). Moreover, anaerobic glycolysis typically is able to use only carbohydrate fuels, produces only a limited total amount of ATP in any one episode, and creates a product, lactic acid, that requires a long time to be cleared from the body.
Anaerobic glycolysis also has great advantages in comparison with aerobic catabolism, however. Because anaerobic glycolysis does not require O
2
, it can provide ATP when O
2
is unavailable, or it can supplement aerobic ATP production when O
2
is insufficient. Of equal importance, anaerobic glycolysis can accelerate very rapidly and reach an exceptionally high rate of ATP production almost instantly. These properties make anaerobic glycolysis indispensible for meeting the ATP needs of burst exercise.
Two Themes in Exercise Physiology: Fatigue and Muscle Fiber Types
During muscular exercise, performance is affected by fatigue and by the fact that a muscle often consists of two or more types of fi-
bers (cells) that differ in their contractile and metabolic properties. Here we discuss these important themes.
Fatigue has many, context-dependent causes
Muscle fatigue
is an exercise-induced reduction in a muscle’s ability to generate peak forces and maintain power output. It is a fascinating and critical physiological phenomenon—having life-
and-death consequences in natural settings—that has defied full understanding. Fatigue clearly has multiple causes, depending on the type and duration of exercise, and on the physiological status of the individual performing the exercise.
The fatigue associated with lactic acid accumulation is one of the types of fatigue that is best understood phenomenologically. Humans or other vertebrates undergoing intense exercise that involves sustained net lactate production characteristically become profoundly overcome with fatigue when lactic acid accumulates to a certain level; virtual paralysis often sets in. Even in the case of this very regular and predictable type of fatigue, however, the cellular and molecular mechanisms are not well understood. In the 1960s and earlier, the lactate ion was considered to be a specific “fatigue factor.” By 1990, however, investigators had decided that the acid–base disturbance associated with lactic acid accumulation—
acidification of muscle cells—was the real cause of this fatigue. By now, acidification—although it plays a role—seems unlikely to be the principal cause, based on studies in which muscle cell pH has Genetic Engineering as a Tool to Test Hypotheses of Muscle Function and Fatigue
BOX 8.2
Experiments based on genetic engi-
neering are increasingly being used to test physiological hypotheses. Genetic engineering methods, for example, are being used extensively to study muscle function and fatigue. One example is provided by research on the role of the phosphagen creatine phosphate
. Be-
cause creatine phosphate in mamma-
lian muscle can be mobilized extremely rapidly to make ATP at a high rate (see Table 8.1), physiologists have long hy-
pothesized that a cell’s phosphagen serves as a principal source of ATP dur-
ing the first seconds of burst exercise. ATP synthesis from creatine phosphate depends on the enzyme
creatine kinase
(
CK
) (see Figure 8.7B). One way to test the hypothesis of phosphagen func-
tion is to lower or raise the levels of CK in muscle cells by genetic engineering methods. If the hypothesized role of creatine phosphate in burst exercise is correct, lowering or raising the levels of CK should interfere with or facilitate burst exercise.
Mutant mice deficient
in CK have been reared by genetic engineering methods. The muscles of these mice clearly exhibit compensatory adjust-
ments that tend to make up for the effects of their CK deficiency (see page 83). Even with such compen-
sations, however, the muscles exhibit a subnormal ability to perform burst activity, and this performance deficit increases with the extent of their CK defi-
ciency. Mice have also been engineered to produce unusually high amounts of CK. Their muscles contract faster than normal muscles in the first moments of isometric twitches. These experiments support the hypothesized role of creatine phosphate in burst exercise.
Box Extension 8.2
discusses another example of the application of genetic engineering methods to the study of muscle, focusing on fatigue.
Creatine kinase (CK)
08_HILL4E.indd 201
4/1/16 1
been directly manipulated to observe its effects. Investigators have not, however, reached a consensus on the actual mechanism. Ac-
cumulation of lactic acid clearly serves as an indicator—a proxy—for the development of this sort of fatigue without lactic acid being the principal causative agent.
14
Some types of fatigue seem to be partly caused by changes in organ-level systems. For example, a type of fatigue that meets this description and is fairly well understood is the fatigue that develops after a long time during intense, sustained
(aerobically fueled) exercise (
FIGURE 8.8
). When people engage in this sort of exercise, fatigue is associated with (and probably partly caused by) inadequate muscle glucose. In the first tens of minutes of such exercise, glucose from muscle glycogen fuels the portion of muscle ATP production that is based on glucose oxidation. As time passes, however, muscle glycogen becomes depleted,
15
and the muscles become dependent on glucose brought to them from other organs (notably the liver) by blood flow (see Figure 8.8). When the rate of this glucose resupply is inadequate, fatigue sets in. Hyperthermia (unusually high body temperature) is another organismal factor that sometimes causes fatigue.
Usually, however, fatigue is caused by changes in cell function and molecular function in the exercising muscles, explaining why the overwhelming focus of modern fatigue research is on cellular-
molecular mechanisms (see Box 8.2). As discussed in Chapter 20, both the excitation of muscle and the contraction of muscle entail ion fluxes across cellular or intracellular membranes mediated by ion transport proteins. Contraction, moreover, entails interactions among the contractile proteins, ATP, and other cellular molecules. Disruption of any of these constituents has the potential to diminish a muscle cell’s ability to develop force and maintain power output. For instance, during the sort of sustained exercise discussed in the last paragraph, part of the cause of fatigue seems to be a gradual accumulation of critical ions (e.g., Ca
2+
) in incorrect cellular loca-
tions as a consequence of the repetitive ion fluxes involved in long-duration muscular effort.
14
Some investigators even argue that lactic acid aids
muscular work, such as through effects on cell-membrane ion channels that help prevent deleterious changes in intracellular and extracellular ion concentrations.
15
Recall (see Figure 6.2
) that carbohydrate stores are small in comparison with fat stores. Even when a person’s glycogen stores are fully loaded, the human body contains only about 450 g (1 pound) of glycogen. The small size of the glycogen stores helps explain why glycogen depletion can occur during prolonged exercise.
The muscle fibers in the muscles used for locomotion are heterogeneous in functional properties
The muscle tissue of an animal is typically het-
erogeneous in its metabolic and contractile properties. In vertebrates, for example, several different types of muscle fibers (muscle cells)
16
occur in skeletal muscle. Two of the principal types in mammals are termed slow oxidative
(
SO
) fibers and
fast glycolytic
(
FG
) fibers. The SO fibers are called slow
because they contract and develop tension relatively slowly, in contrast to the FG fibers, which are fast
in contracting and developing tension (see Table 20.2). The SO fibers are called oxidative
, whereas the FG fibers are called glycolytic
, because of dif-
ferences in the physiology of their ATP production. The SO fibers are poised principally to make ATP by aerobic catabolism; they have high levels of key enzymes specific to aerobic catabolism, such as succinic dehydrogenase (a Krebs-cycle enzyme), and are well endowed with mitochondria. The FG fibers, by contrast, are poised principally to make ATP anaerobically; they are high in enzymes of anaerobic glycolysis, such as lactate dehydrogenase, and are relatively sparse in mitochondria.
Correlated with the differences in the catabolic pathways they use, the SO and FG fibers differ in how readily they can take up and store O
2
. SO fibers are relatively rich in myoglobin, which not only helps store O
2
within the fibers but also aids diffusion of fresh O
2
into the fibers. FG fibers are low in myoglobin. Because of the difference in myoglobin content, SO fibers are reddish in color, whereas FG fibers are whitish, explaining why the SO fibers are sometimes called red
fibers, and the FG fibers white
.
SO and FG fibers also differ in their power-generation and fatigue properties in ways that correlate with the features already discussed. The properties of SO fibers are predictable from the fact that they mainly use steady-state aerobic catabolism to make ATP: Although SO fibers have relatively low peak mechanical-power outputs (see Table 8.1), they are relatively resistant to fatigue and readily sustain work over long periods. FG fibers represent the opposite extreme. They can generate a high power output, but they rely strongly on nonsteady-state mechanisms of ATP production, accumulate lactic acid, and fatigue quickly.
In mammals, major skeletal muscles are typically built of mixes of intermingled SO fibers, FG fibers, and other fiber types (see Chapter 20). Fish, by contrast, often have large muscle masses composed principally of a single type of muscle fiber similar to the mammalian SO or FG fibers; thus entire blocks of muscle in fish are red or white and exhibit the performance properties of red or white fibers.
16
A muscle fiber is a muscle cell. “Fiber” and “cell” are synonyms in the study of muscle.
FIGURE 8.8 The fueling of intense, sustained muscular work in humans Shown are the fuels that the muscles use to make ATP over the course of 4 h of exercise that requires about 70%–80% of a person’s maximum rate of aerobic catabolism. In the later stages of this exercise, a failure of glucose resupply pro-
cesses to keep up with muscle needs is believed to be an important cause of fatigue; the resupply processes include mobilization of liver glucose and blood transport to muscles. (After Coyle 1991.)
At each time, the percentage of the total vertical distance that falls within each category of fuel corresponds to the percentage of ATP made from that fuel.
As time passes, muscle glycogen is depleted, meaning that…
…glucose must be brought to
the muscles at an increasing rate
from elsewhere in the body.
Percentage of ATP made from various fu
Fatty acids brought to muscles by blood
Number of hours after start of exercise
Glucose from glycogen stored in exercising muscles
Glucose brought to muscles by blood
80
60
40
20
0
0
1
4
2
3
08_HILL4E.indd 202
4/1/16 1
The Interplay of Aerobic and Anaerobic Catabolism during Exercise
When animals engage in exercise, their performance typically reflects the underlying mechanisms that they are using to produce the ATP required. Fish that are cruising about at relatively leisurely speeds, for example, do so with their red swimming muscles, employing steady-state aerobic catabolism to make ATP. Cruising, therefore, can be sustained for long periods. However, fish that engage in sud-
den, intense exertion—such as cod avoiding a trawling net or salmon leaping waterfalls—use their white swimming muscles and anaerobic glycolysis to generate the high power they require.
17
They thus accu-
mulate lactic acid, and if they must perform repeatedly in a short time, they are in danger of the sort of fatigue associated with lactic acid.
Crustaceans similarly illustrate that exercise performance reflects the underlying mechanisms of ATP production. When crayfish, lobsters, and crabs walk or cruise about at modest, sustainable speeds, they produce ATP by steady-state aerobic catabolism. When a crayfish or lobster employs tail flipping to power itself rapidly away from danger, however, the tail muscles require ATP at a greater rate than aerobic catabolism can provide. Thus the muscles turn to mechanisms that can produce ATP at exceptionally high rates. In species of crayfish studied in detail, the tail muscles exhibit high levels of arginine kinase and lactate dehydrogenase—enzymes instrumental in anaerobic ATP production—and low levels of aerobic catabolic enzymes. The primary mechanism of ATP production at the start of escape swimming by tail flipping is use of the phospha-
gen arginine phosphate. Later, if tail flipping continues, anaerobic glycolysis—producing lactic acid—is brought into ever-greater play to meet the ATP demands of the tail muscles. Because both of these mechanisms are nonsteady-state and self-limiting, tail flipping cannot be sustained for long.
A huge amount of information exists on lactic acid concentra-
tions in relation to fatigue. Although lactic acid is not the cause
of fatigue, it is nonetheless often a useful, readily measured index
of cell conditions causing fatigue. As a practical index, animals are often considered to have an upper limit of lactic acid accumula-
tion. According to this view, after one bout of exercise has caused a buildup of lactic acid, a closely following second bout is limited in the degree to which anaerobic glycolysis can be used because newly made lactic acid adds to the preexisting amount. A human example is provided by athletes who compete in two burst-type races in a single track meet; performance in the second race is often impaired if lactic acid accumulated in the first race has not yet been fully metabolized. Clearing lactic acid from the body takes tens of minutes in mammals and can take hours in poikilotherms. For animals in nature, the slow rate of removal of lactic acid may well mean that an animal is impaired for a substantial time in its ability to pursue prey or escape danger. These important behavioral consequences are additional manifestations of the high degree of relevance of the biochemistry of ATP production. Some types of animals follow patterns that are different from those of vertebrates and crustaceans. Insects are the most dramatic 17
When we speak of red and white muscles in this context, we are referring to the distinction between the myoglobin-rich, SO-like muscles and the myoglobin-poor, FG-like muscles discussed in the previous section. This is an entirely different matter from the overall hue of a fish’s muscle tissue, whether off-white in a cod or orange in a salmon.
and important example. Insect flight muscles typically have little or no ability to make ATP anaerobically and remain fully aerobic even when they suddenly increase their power output, as at the onset of flight. Correlated with the fact that virtually all flight-muscle work is aerobic, insect flight muscles contain very high levels of aerobic catabolic enzymes, and in some species, half the tissue volume of these muscles is mitochondria! The flight muscles of certain insects, in fact, are the most aerobically competent of all animal tissues as judged by the rate per gram at which they can synthesize ATP by aerobic catabolism. A characteristic of insects that helps explain their aerobic fueling of burst exercise is their tracheal breathing system, which provides O
2
directly to each flight-muscle cell by way of gas-filled tubes (see page 629).
When squids and other molluscs use anaerobic catabolism for burst swimming, they exhibit a curious difference from vertebrates. Their swimming muscles reduce pyruvic acid to octopine
rather than lactic acid. The octopine-generating anaerobic pathway provides a high rate of ATP synthesis, as in vertebrates, but has different detailed biochemical implications.
Underlying much of scientific thinking about exercise in the wild is an assumption that a high capacity for exercise performance is advantageous for survival and reproduction—meaning that a high capacity for performance is favored by natural selection. Although some tests of this hypothesis have not obtained positive results, direct evidence is in fact accumulating that, in a state of nature, individual animals of a single species vary in exercise performance and this variation affects their survival and reproductive success as hypothesized. An example is provided by male collared lizards (
Crotaphytus collaris
) that are defending territories where they mate with females. To defend these territories the males employ sudden, high-speed running to exclude intruders and meet other threats. Their burst running almost surely depends on ATP produced by anaerobic glycolysis because (see Table 8.1), in such running, they accelerate almost instantly and achieve high work intensities. Individual males vary substantially in how fast they can cover distance during burst running, from about 3 to 5 m/s. Investigators measured the burst running speeds of 16 males in a free-living population. Then they used genetic paternity testing to determine the number of offspring fathered by each. They found a statistically significant trend for the faster males to father more offspring (
FIGURE 8.9
). Number of offspring fathered
3.0
2.5
3.5
4.0
4.5
5.0
Burst speed (m/s) on a log scale
0
2
4
6
8
10
12
FIGURE 8.9 Modern genetic paternity testing reveals that the number of offspring fathered by male collared lizards (
Crotaphytus collaris
) depends directly on how fast they can run during burst running Each symbol represents one male in a free-
living population. Males defend territories where they mate with females. The defense of a territory depends in part on sudden, high-intensity running to repel male invaders or meet other threats. (After Husak et al. 2006.)
08_HILL4E.indd 203
4/1/16 1
Your preview ends here
Eager to read complete document? Join bartleby learn and gain access to the full version
- Access to all documents
- Unlimited textbook solutions
- 24/7 expert homework help
Metabolic transitions occur at the start and end of vertebrate exercise
Multiple metabolic processes are involved in the provision of ATP for vertebrate exercise of all types. Details depend on the intensity of exercise, which is usefully indexed by comparison with an individual’s maximum rate of aerobic catabolism. A given individual in a particular state of train-
ing is capable of a certain maximum rate of O
2
consumption.
18
Exercise that requires exactly this maximum is classified as maximal exercise
. Exercise that requires less than the maximum rate of O
2
consumption is called submaximal exercise
, and exercise that requires more than an individual’s maximum rate of O
2
consumption is called supramaximal exercise
.
Consider a bout of submaximal exercise that starts and ends abruptly, requires about 80% of an individual’s maximum rate of O
2
consump-
tion, and lasts 30 min or so. Let’s assume we are talking about a person who is running, although the principles we will develop apply to all or most vertebrates. FIGURE 8.10A
shows how the person’s rate of O
2
consumption by breathing would change during this bout of exercise if all
ATP were made on a moment-by-moment basis by aerobic catabolism using O
2
taken up from the environment by breathing. The person’s rate of O
2
consumption would increase stepwise at the start of running and decrease stepwise at the end.
Actually, however, during this type of exercise, a person’s rate of O
2
uptake by breathing changes as shown by the red line in FIGURE 8.10B
. In the middle of the bout of exercise, the person’s actual rate of O
2
uptake matches the theoretical O
2
demand of the exercise. However, there is a transition phase at the start of the exercise, when the person’s actual rate of O
2
uptake is lower than the theoretical O
2
demand, and there is another transition phase at the end when the person’s actual rate of O
2
uptake exceeds the theoretical O
2
demand.
THE TRANSITION PHASE AT THE START: THE OXYGEN DEFICIT The reason for the transition phase at the start of the exercise we are considering is the fact, already mentioned, that the breathing and circulatory systems in vertebrates do not instantly increase the rate at which they deliver O
2
to the body. Instead, even if ex-
ercise
starts abruptly
, O
2
delivery
to the tissues increases gradually
. In people, 1–4 min are required for the breathing and circulatory systems to accelerate fully. During the period when the breathing and circulatory systems are accelerating their actual rate of O
2
delivery at the start of a bout of exercise, the body’s supply of O
2
from the environment (actual O
2
uptake) is less than its theoretical O
2
demand for the exercise. This difference is termed an oxygen deficit
(see Figure 8.10B).
18
See Chapter 9 for an extensive discussion of the maximum rate of O
2
consumption and its determinants.
During the period of the oxygen deficit, the full ATP demand of exercise is not
met by aerobic catabolism based on environmental O
2
. Where, then, does the other ATP come from? In the sort of exercise we are discussing, the answer is that ATP is contributed during the period of oxygen deficit by anaerobic glycolysis, use of phosphagen, and use of O
2
stores.
19
These three mechanisms, in fact, are
essential for exercise to start in a stepwise way. They make up for the slow acceleration of ATP production by aerobic catabolism based on environmental O
2
. They thereby permit the overall
rate of ATP production to increase abruptly to a high level when exercise begins.
THE PAY-AS-YOU-GO PHASE During the exercise we are discuss-
ing or any other sort of submaximal exercise, the breathing and circulatory systems ultimately accelerate their rate of O
2
delivery sufficiently to meet the full O
2
demand of the exercise. The exercise is then said to enter a pay-as-you-go phase
(see Figure 8.10B) 19
Anaerobic glycolysis, use of phosphagen, and use of O
2
stores are sometimes termed the
mechanisms of oxygen deficit
. …and decrease stepwise at the end.
Under the highly theoretical assumption that all
ATP is made aerobically using environmental O
2
,
a person’s rate of O
2
uptake by breathing would
increase stepwise at the start of exercise…
In real exercise, the difference between the
theoretical O
2
demand and the actual O
2
uptake at the start is the oxygen deficit
, and…
…the difference between theoretical O
2
demand and actual O
2
uptake at the end is the excess
postexercise oxygen consumption (
EPOC
)
.
all ATP is made using O
2
from the atmosphere
Rate of theoretical
O
2
demand
(B) Actual rate of O
2
consumption compared with theoretical
Rest
Rest
Exercise
Time
Time
Rate of O
2
demand or supply
Pay-as-you-go phase
Actual O
2
uptake from environment
Theoretical O
2
demand
Resting rate of O
2
consumption
FIGURE 8.10 The concepts of oxygen deficit and excess postexercise oxygen consumption (A) The rate of O
2
demand of a person who is initially at rest, suddenly starts vigorous submaximal exercise, continues for 30 min or so, and then suddenly stops, assuming unrealistically that all ATP is produced aerobically, using atmospheric O
2
, at all times. (B) The actual rate of O
2
uptake from the environment (red line) of the person in (A), showing that there is an initial transition period during which the full ATP demand is not met by O
2
uptake, then a period when O
2
uptake matches the full O
2
demand, and finally a transition period when the person’s actual rate of O
2
uptake exceeds the resting rate even though the person is at rest.
08_HILL4E.indd 204
4/1/16 1
because thereafter its full O
2
cost is met on a moment-to-moment basis by use of O
2
taken up from the environment by breathing. With all ATP being made by steady-state aerobic catabolism, the exercise can in principle be sustained indefinitely.
The pay-as-you-go phase in submaximal exercise begins soon enough, in general, to prevent biochemical self-termination of anaerobic glycolysis, use of phosphagen, and use of O
2
stores. Al-
though those three mechanisms are susceptible to self-termination, they are not called upon for long enough in this sort of exercise to reach their inherent limits.
THE TRANSITION PHASE AT THE END: EXCESS POSTEXERCISE OXYGEN CONSUMPTION At the end of the exercise we are describing, the exercising person suddenly stops running, but thereafter his or her actual rate of O
2
uptake does not suddenly drop in stepwise fashion. Instead, it declines gradually, remaining above the resting rate of O
2
consumption for many minutes (see Figure 8.10B). This elevation of the actual rate of O
2
uptake above the resting rate of O
2
uptake—even though the person is behavior-
ally at rest—is termed excess postexercise oxygen consump-
tion
(
EPOC
). In everyday language, people describe the EPOC as “breathing hard” after exercise.
20
The EPOC has multiple causes, and the reasons for it are not fully understood at present.
20
For several decades in the mid-twentieth century, the EPOC was called oxygen debt
. That term has been discredited, however, because it was based on an assumption that the cause of the EPOC is simply the need to metabolize any lactic acid that was accumulated at the start of the exercise. Actually, there is often a nearly total lack of correspondence between the time course of the EPOC and the time course of lactic acid metabolism in vertebrates. The term EPOC
is preferred over
oxygen debt
because it is functionally neutral and implies no particular mechanistic explanation.
THE TRANSITION PHASES VARY IN THEIR NATURE DEPENDING ON THE INTENSITY OF EXERCISE Different intensities of exer-
cise in vertebrates produce different sorts of transition phases. For example, if a vertebrate undertakes light submaximal
exercise that requires less than 50%–60% of the individual’s maximum rate of O
2
consumption, transition phases occur at the start and end, as shown in FIGURE 8.11A
, but lactic acid does not accumulate at these exercise intensities. Without a lactic acid accumulation, the only processes required to “reinitialize” the body at the end of exercise are the replenishing of O
2
stores and phosphagen stores, both of which occur very rapidly (see Table 8.1).
In heavy submaximal exercise that requires more than 50%–60% of maximum O
2
consumption (
FIGURE 8.11B
), net accumulation of lactic acid occurs at the start. Accordingly, lactic acid must be metabolized at the end—a long process. The EPOC therefore lasts longer than in light submaximal exercise.
The most dramatic transition phases occur during supramaximal exercise (
FIGURE 8.11C
). Such exercise demands ATP at a greater rate than can ever
be supplied by steady-state aerobic catabolism. Thus a pay-as-you-go phase is never reached, and anaerobic glycolysis must continue to be tapped for ATP for as long as the exercise continues—causing a steadily increasing oxygen deficit and buildup of lactic acid. Supramaximal exercise is the principal form of exertion in which lactic acid accumulates to such high levels that the profound, debilitating, “lactic acid type” of fatigue can occur
. In fact, unless an individual electively stops exercising, supramaximal exercise typi-
cally undergoes metabolic self-termination within minutes. Then, associated with the large accumulation of lactic acid, a long time is required for the body to recover, during which the individual’s capability for further supramaximal exertion is impaired.
(A) Light submaximal exercise
Maximal rate of O
2
consumption
Rate of O
2
demand or supply
Time
Time
Time
(B) Heavy submaximal exercise
Rate of O
2
demand or supply
Rate of O
2
demand or supply
(C) Supramaximal exercise
KEY
Theoretical O
2
demand
Actual O
2
uptake from environment
Oxygen deficit
Excess postexercise oxygen consumption
FIGURE 8.11 Stylized O
2
supply–demand diagrams for light sub-
maximal, heavy submaximal, and supramaximal exercise The format is as in Figure 8.10. The patterns illustrated are those observed in mammals. Two important determinants of exercise performance are an individual’s maximum rate of O
2
consumption and maximum oxygen deficit. Both are increased by training (repeated exercise), thereby increasing per-
formance. A person, for example, might increase his or her maximum rate of O
2
consumption by 10%–30% through appropriate training.
08_HILL4E.indd 205
4/1/16 1
The ATP source for all-out exercise varies in a regular manner with exercise duration
If you consider human competitive running and reflect on the progression from a sprint to a mile race to a marathon, you will notice that as the duration of all-out exertion increases, the pace slows. Mile races are run slower than sprints, and marathons are run slower than mile runs. This trend in performance, which is a fairly general property of animal exercise, is a
direct reflection of the biochemistry of ATP production.
In a person or other vertebrate, when all-out exertion lasts 10 s or so, the total amount of ATP needed, from start to finish, is relatively small (simply because ATP must be provided for only a short time). This means that anaerobic glycolysis, phosphagen, and O
2
stores can in principle meet the full ATP requirement. In actual practice, when people run the 100-yard dash (or 100-m dash), these three mechanisms of ATP production meet at least 90% of the ATP cost; some champions hold their breath from start to finish! Anaerobic glycolysis and the use of phosphagen and O
2
stores—while unable to make a great quantity of ATP—are able to produce ATP at exceptionally high rates
(see Table 8.1). Thus, when these mechanisms are sufficient to meet most of the cost of running, the pace of running can be very fast, as it is in the 100-yard dash.
In a mile race (about the same as a 1500-m race), the total ATP requirement is much greater than in a 100-yard dash. Even if anaerobic glycolysis, phosphagen, and O
2
stores are fully exploited to make as much ATP as they possibly can during a mile race, they can meet no more than 25%–50% of the total ATP need. The rest of the ATP must be made by steady-state aerobic catabolism using O
2
from the environment, a mechanism that cannot produce ATP as fast as the other mechanisms (see Table 8.1). Thus, taking into account the rates of all the processes that are required to contribute ATP during a mile race, the overall rate of ATP production is lower than that during a 100-yard dash. The pace of the mile race must therefore be slower. The pace of a marathon must be slower yet because the total ATP requirement of a marathon is so great that only 2%–3% of it can be met by use of anaerobic glycolysis, phosphagen, and O
2
stores. In a marathon, almost all
of the ATP is made by steady-state aerobic catabolism, limiting the pace to that which is permitted by that mechanism.
FIGURE 8.12
shows how the mechanisms of ATP production vary with the duration of exertion in world-class competitive runs. The red line depicts the speed of running as a function of duration. The subdivision of the space below the red line shows how ATP is made. With increasing duration, ATP production shifts from being principally anaerobic (based on phosphagen and anaerobic glycolysis) to being chiefly aerobic. Moreover, at marathon and ultramarathon distances, aerobic catabolism shifts from exclusive use of carbohydrate fuels toward substantial use of lipid fuels, which permit only a lower rate of ATP synthesis than carbohydrate fuels (see Table 8.1).
Trends similar to those in Figure 8.12 apply to vertebrates living in the wild. Thus ecologically relevant performance depends in a regular way on the biochemical mechanisms by which ATP is made. As the primary mechanism of ATP synthesis shifts from phosphagen use to anaerobic glycolysis and then to aerobic catabolism based on carbohydrate and lipid fuels, the pace slows.
Related species and individuals within one species are often poised very differently for use of aerobic and anaerobic catabolism
Related species sometimes have evolved very different emphasis on aerobic and anaerobic ATP production during intense exertion, and these differences can have important life-history consequences. Thus the biochemistry of ATP production is one of the ways that species become specialized to live as they do.
The terrestrial amphibians we briefly noted in Chapter 2 (see Figure 2.10) provide a classic illustration. Some species, such as the common leopard frog (
Rana pipiens
) and many other ranid and hylid frogs, have a muscle biochemistry that emphasizes anaerobic glycolysis as the principal mechanism of ATP production during all-out exertion. If you chase a leopard frog, at first the frog flees by jumping away very rapidly, but within a few minutes it col-
lapses in fatigue. Both the high speed of the initial jumping and The fraction of the vertical distance between the red line and the x
axis that falls within each category of ATP production corresponds to the fraction of ATP made by each mechanism of production.
…essentially all ATP is made by aerobic catabolism in long-duration events.
Most ATP is made by anaerobic
glycolysis and use of phosphagen in short-duration events, whereas…
200 m
800 m
400 m
100-m dash
ATP from anaerobic glycolysis
ATP from
phosphagen
Duration (s) on log scale
ATP from aerobic catabolism using glycogen and glucose
Marathon
(42,194 m)
10,000 m
5000 m
1500-m run
100,000 m
50
10
500
5000
10,000
1000
100
Red line: Average speed (m/s)
2
0
4
6
8
10
ATP
from aerobic
catabolism using lipid
Speed of running in event specified
FIGURE 8.12 The mechanisms of meeting the ATP costs of world-class competitive running The red line shows the average pace of races as a function of the length of time the pace is maintained, based on world records for men in the specified competitive events. The space below the red line shows the approximate fraction of ATP made by aerobic catabolism, anaerobic glycolysis, and use of creatine phosphate. For simplic-
ity, synthesis of ATP by use of O
2
stores is not shown as a separate category. (Data on ATP production after Newsholme et al. 1992.)
08_HILL4E.indd 206
4/1/16 1
Your preview ends here
Eager to read complete document? Join bartleby learn and gain access to the full version
- Access to all documents
- Unlimited textbook solutions
- 24/7 expert homework help
the quick fatigue reflect the emphasis on anaerobic glycolysis to make ATP. Many of the bufonid toads, such as the western toad (
Anaxyrus boreas
, formerly Bufo boreas
), exemplify the opposite extreme. The toads have a muscle biochemistry that emphasizes aerobic production of ATP during all-out exertion. When chased, they do not flee as quickly as leopard frogs, but they can continue jumping at a steady pace for a long time. Both their slow speed and their resistance to fatigue reflect the aerobic fueling of their exercise. Lactate dehydrogenase (LDH) represents a key respect in which the frogs and toads differ; the leg muscles of the frogs express LDH at higher concentration than those of the toads.
A parallel, and very dramatic, example is provided by species of fish. The skipjack tuna (
Katsuwonus pelamis
) is a supremely vigorous species of fish. The enzymatic activity of LDH in its white swim-
ming muscles exceeds the activity of LDH in sluggish fish species by more than 1000 times.
21
The tuna is thereby able to engage in exceptionally intense burst exercise.
At a different scale, individuals within a single species are often poised differently for use of aerobic and anaerobic catabolic pathways. A particularly intriguing example of this sort of varia-
tion is that humans vary widely in the fiber composition of their muscles, as illustrated in FIGURE 8.13
. The swimmer at the top competes in sprints that require intense exertion for short periods. The cyclist, however, competes in sustained cycling races. Small pieces of tissue were removed from identical thigh muscles of the two men and subjected to a histochemical procedure that darkly stains slow oxidative (SO) muscle fibers. The images to the right in Figure 8.13 show that few of the muscle fibers of the swimmer are SO fibers, whereas most of the fibers of the cyclist are SO fibers. Most researchers believe that a difference of this specific sort (percentage of SO fibers) is relatively fixed. That is, people like the cyclist, it is thought, have principally SO fibers from early childhood. Such people discover through experience that their muscles, although not extremely powerful, are resistant to fatigue; and if such people are inclined toward athletic competition, they choose sports such as long-distance cycling that are well served by SO muscle fibers. In contrast, people like the swimmer, whose muscles are low in SO fibers and presumably high in fast glycolytic (FG) fibers, gravitate toward sports such as sprint swimming in which high power—rather than long-term endurance—is a key to success.
21
Enzyme activity
is measured as the maximum rate at which substrate can be converted to product by a unit weight of tissue.
SUMMARY
The Interplay of Aerobic and Anaerobic Catabolism during Exercise
Behavior and biochemistry are linked during physical activity because attributes of performance depend on how the ATP for muscular effort is synthesized.
Submaximal forms of exercise can be supported entirely (except during transition phases) by aerobic catabolism using O
2
taken in from the environment by breathing. From the viewpoint of ATP supply and demand, submaximal forms of exercise can thus be sustained indefinitely.
Supramaximal forms of exercise in vertebrates, crustaceans, and some other animals require a continuing input of ATP from anaerobic glycolysis. The steady use of anaerobic glycolysis—manifested by a steady accumulation of lactic acid—eventually causes metabolic self-termination of the exercise.
In vertebrates, metabolic transitions occur at the start and the end of even light submaximal exercise. An oxygen deficit occurs at the start, and excess postexercise oxygen consumption (EPOC) occurs at the end. The oxygen deficit is a consequence of the fact that the breathing and circulatory systems increase O
2
delivery gradually, not stepwise, at the start of exercise.
As the duration of all-out exertion increases, ATP must increasingly be supplied by steady-state aerobic catabolism, rather than by nonsteady-state mechanisms that can produce ATP exceptionally rapidly but cannot produce a great deal of it. The pace of all-out exertion therefore declines as duration increases.
Closely related species, and even individuals within one species, often differ greatly in their emphasis on aerobic and anaerobic mechanisms of producing ATP for exercise. These metabolic differences help explain differences in exercise performance.
(B) tion of their thigh muscles (A) The swimmer competes in 50-m sprints. (B) The cyclist competes in long-distance races. Shown to the right of each man is a microscopic section of his vastus lateralis
, a thigh muscle, stained to make slow oxidative (SO) fibers dark. The sec-
tions are labeled with a different nomenclatural system from the one we use in this chapter: I = SO fibers; II = either FG fibers or other fast-
contracting fibers. (From Billeter and Hoppeler 1992; courtesy of Rudolf Billeter-Clark and Hans Hoppeler.)
08_HILL4E.indd 207
4/1/16 1
Responses to Impaired O
2
Influx from the Environment
In addition to vigorous exercise, impaired O
2
influx from the envi-
ronment is the second major reason that animals turn to anaero-
bic catabolic pathways to make ATP. Many animals experience reduced O
2
influx from their environments during parts of their lives. The situation can arise in two ways. First, O
2
influx may be reduced because the concentration of O
2
in the environment is low. Alternatively, animals may enter environments in which they cannot breathe, as when seals or whales dive. Under either set of conditions, it is common for at least some tissues to experience hypoxia
or anoxia
, defined respectively to be an especially low level of O
2
in tissues or an absence of O
2
in tissues.
Some animals display metabolic depression when they are faced with reduced O
2
influx. Metabolic depression
is a regulated reduction in the ATP needs of the animal (or certain of its tissues) to levels below the needs ordinarily associated with rest in a way that does not present an immediate physiological threat to life. When deprived of O
2
, for example, brine shrimp embryos switch to a greatly reduced rate of metabolism (signifying a reduced rate of ATP use), and they are able to sustain this state for long periods and later recover without ill effect (
FIGURE 8.14
). Study of the mechanisms of metabolic depression is in its infancy. In some cases, key, rate-limiting enzymes are downregulated, or numbers of mitochondria decline. The hypoxia-inducible factor 1 (HIF-1) control system is often involved (see Figure 23.6), as are other control systems.
To make ATP during anoxia, animals must turn to anaerobic catabolic pathways, and they also often use such pathways to help make ATP during hypoxia. When vertebrates turn to anaerobic catabolism, they almost universally produce lactic acid,
22
which they never excrete. In sharp contrast, invertebrates adapted to life without influx of O
2
rarely produce lactic acid. Instead, they usually employ anaerobic catabolic pathways that are more elaborate than anaerobic glycolysis and that yield a variety of different products, which often are excreted. All anaerobic catabolic pathways produce far less ATP per food molecule than aerobic catabolism does. Thus, when animals turn from aerobic to anaerobic catabolic pathways as their means for long-term ATP production, they are less able to make ATP. Metabolic depression helps with this situation because it reduces the rate at which animals need
ATP.
Air-breathing vertebrates during diving: Preserving the brain presents special challenges
Most vertebrate brains are obligatorily aerobic; they must have O
2
. When air-breathing vertebrates dive for extended periods, therefore, the status of their brain is of special interest and concern.
In diving species of mammals and birds, most dives are kept short enough that the ATP needs of all tissues can be met aerobically using stored O
2
. Dives of exceptionally long duration, however, cause these vertebrates to resort to anaerobic glycolysis. The animals then metabolically subdivide their bodies (see Chapter 26 for details). They ensure continuing O
2
delivery to their brain by reserving certain of their O
2
stores for the brain, while simultaneously they deprive large portions of their bodies of O
2
delivery; the latter parts run out of O
2
and become dependent on anaerobic glycolysis. Diving crocodilians, sea turtles, lizards, and most freshwater and terrestrial turtles must also keep their brains aerobic.
Certain species of freshwater and terrestrial turtles, however, are dramatic exceptions in that they can tolerate total-body an-
oxia—
full O
2
depletion of all tissues, including the brain
—during protracted dives. These anoxia-tolerant turtles are able to dive for exceptionally long periods. FIGURE 8.15
shows the lengths of time that various vertebrates can survive anoxia as a function of their body (tissue) temperatures when they are tested. The anoxia-tolerant turtles (in which the brain can survive without O
2
), such as those in the genera Trachemys
and Chrysemys
, are able to survive about 1000 times longer at any given body temperature than most other vertebrates.
An informative way to understand the implications of brain anoxia in diving turtles is to look briefly at the threat anoxia poses to vertebrate central nervous system (CNS) tissue. In a person or other mammal, catastrophe strikes when the brain is deprived of O
2
because the ATP requirement of mammalian brain cells per unit of time far exceeds the rate at which those cells can make ATP by anaerobic means. Within seconds
after O
2
influx to the mammalian brain is cut off, the concentration of ATP in brain cells starts to fall precipitously. Soon, ATP-dependent ion pumps (e.g., Na
+
–K
+
-ATPase) are unable to pump ions across the cell membranes rapidly enough to maintain normal membrane po-
larization. The cell membranes thus depolarize, with numerous cataclysmic consequences; for instance, nerve impulses (action potentials) become impossible, and voltage-gated Ca
2+
channels are inappropriately triggered to open, allowing Ca
2+
to flood into the cells. For this reason and others, the Ca
2+ concentration in the 22
A few vertebrate species, notably two species of fish discussed later, are exceptions.
Brine shrimp embryos enter a sustained state of profound metabolic depression when denied O
2
.
Return to O
2
No O
2
Rate of heat production (mJ/g
•
s)
8
4
4
6
0
2
2
10
12
14
16
6
8
Time from start of experiment (h)
Ammonia
FIGURE 8.14 Metabolic depression in an invertebrate faced with anoxia Metabolic rate was quantified directly, by measure-
ment of heat production. Two groups of embryos of brine shrimps (
Artemia
)—represented by the two lines—were studied. They were living in water near equilibrium with the atmosphere at the start of the experiment. Then, at the time marked “No O
2
,” they were switched into O
2
-free water. The embryos were exposed to ammonia while in O
2
-free water, as a way of raising their pH. Their response to ammonia supports the hypothesis that metabolic depression in the embryos is partly dependent on a low pH. The drawing shows an adult brine shrimp. (After Hand and Gnaiger 1988.)
08_HILL4E.indd 208
4/1/16 1
cytoplasm rises to levels that trigger a variety of inappropriate and disastrous Ca
2+
-mediated responses.
The turtles that tolerate brain anoxia employ metabolic depression of the brain as a key mechanism of maintaining the integrity of their brain tissue during anoxia. Synaptic transmis-
sion between brain cells is suppressed in the absence of O
2
, and ion-mediated bioelectrical activity of cells is reduced so that the brain becomes electrically relatively silent. This response has a significant cost: The turtles cease to be behaviorally alert. They become comatose. Because of this response, however, the brain ion pumps have much less work to do to maintain normal ion distributions and cell-membrane polarization. The ATP requirement of maintaining tissue integrity is lowered, anaerobic glycolysis is able to meet the ATP requirement, and brain ATP concentrations do not fall. With its entire body anoxic, a turtle accumulates lactic acid, which can reach extraordinary concentrations during prolonged anoxia. The shell and the bones of a turtle play key roles in preventing lethal acidification under these circumstances, by buffering the acid.
23
Animals faced with reduced O
2
availability in their usual environments may show conformity or regulation of aerobic ATP synthesis
When animals are living in the environments in which they can breathe, such as fish in water or mammals in air, how do their rates of aerobic ATP production change when they are confronted with changes of O
2
concentration in the environmental water or air? As the environmental O
2
level is lowered, the usual pattern is for an animal’s rate of O
2
consumption to be unaffected over a certain range of O
2
levels (
FIGURE 8.16A
). This maintenance of a steady 23
A parallel phenomenon has recently been discovered in certain crayfish, in which the shell buffers lactic acid produced when the animals are placed in air, where they cannot breathe.
rate of O
2
consumption (and aerobic ATP production) regardless of the level of O
2
in the environment is termed oxygen regulation
(see Figure 1.8). It often involves active responses, such as an in-
crease in breathing rate as the O
2
level in the water or air declines. Ultimately, if the environmental O
2
level is lowered further and 100
1000
10,000
100,000
Anoxic survival time (min) on log scale
0
10
20
30
40
Body temperature (°C) 10
0
Trachemys
turtle
Chrysemys
turtle
Crucian carp
Blind goby
Mouse
Anole lizard Rainbow trout
Bullhead catfish
Anoxia-tolerant
vertebrates
“Normal” vertebrates
KEY
FIGURE 8.15 Survival times during total-body anoxia in verte-
brates as a function of body temperature The survival times are plotted on a logarithmic scale. (After Nilsson and Lutz 2004.)
The redline darter
inhabits fast-
flowing streams. Exposure to O
2
concentrations less than 50% of that of fully aerated water can be fatal.
The slack-water darter
resides in slow-moving streams and is much more tolerant of low O
2
concentrations in its environment.
(B) Regulation and conformity in two species of related fish
O
2
concentration of environmental water or air
Oxygen regulation
Oxygen conformity
(A) The concepts of oxygen regulation and conformity
Rate of O
2
consumption
Routine rate of O
2
consumption (μL/g
•
h)
Water O
2
concentration as percentage of fully aerated
0
25
75
100
50
0
0
30
30
60
60
90
90
120
120
150
180
FIGURE 8.16 Oxygen regulation and conformity (A) The general concepts of oxygen regulation and conformity. (B) Rates of O
2
consump-
tion of two species of fish in the genus Etheostoma
—the redline darter (
E. rufilineatum
) and the slack-water darter (
E. boschungi
)—during routine activity at 20°C. Red circles mark ambient O
2
levels at which deaths occurred. On the x
axis, 100 is the O
2
concentration of water that is at equilibrium with the atmosphere and therefore fully aerated; values higher than 100 were created in some studies by bubbling water with pure O
2
. (After Ultsch et al. 1978.)
08_HILL4E.indd 209
4/1/16 1
Your preview ends here
Eager to read complete document? Join bartleby learn and gain access to the full version
- Access to all documents
- Unlimited textbook solutions
- 24/7 expert homework help
further, oxygen regulation can no longer continue. Instead, the rate of O
2
consumption starts to fall as the environmental O
2
level falls. This condition is termed oxygen conformity
(see Figure 8.16A). High altitudes are an intriguing situation in which these concepts apply to human beings (
BOX 8.3
).
When related species are compared under similar test conditions, their abilities for oxygen regulation and conformity often correlate with the types of habitats in which they live. These abilities therefore seem to have evolved in parallel with habitat selection. An illustra-
tion is provided by two species of related freshwater fish living in a single Alabama watershed (
FIGURE 8.16B
). One species, the redline darter, occurs in fast-flowing streams where O
2
levels tend to be consistently high because turbulence promotes aeration. The other species, the slack-water darter, is found in slow-moving streams where the O
2
concentration may be only one-third as high as in fully aerated water. As Figure 8.16B shows, the slack-water darter, which often experiences low-O
2
waters, exhibits a much broader range of oxygen regulation than the redline darter, which rarely must cope with low O
2
levels. Indeed, lowering the concentration of O
2
to 40%–50% of the fully aerated level causes a sharp depres-
sion of O
2
uptake and deaths in redline darters, whereas it does not affect the O
2
uptake of slack-water darters at all.
Water-breathing anaerobes: Some aquatic animals are capable of protracted life in water devoid of O
2
O
2
-free microenvironments are far more common in bodies of water than in terrestrial environments (see Chapter 22), and biologists have discovered quite a few cases of water-breathing aquatic ani-
mals that can live in O
2
-free settings because they can function as anaerobes
, meaning they are able to survive whole-body anoxia for long periods. Some of the best-studied examples among inver-
tebrates are certain species of clams, mussels, and other bivalve molluscs. Ribbed mussels (
Geukensia demissa
), for example, live in the mud of salt marshes, where they can become buried; they are able to survive in an atmosphere of pure N
2
for 5 days. Certain marine clams that live in seas prone to O
2
depletion, such as the ocean quahog (
Arctica islandica
), can live for 1–2 months in O
2
-free water. Worms and other invertebrates that live at the bottoms of lakes or ponds are other animals that may experience severe and prolonged O
2
deprivation (see Figure 1.16), and some are among the most tolerant to anoxia of all free-living animals. Certain an-
nelid worms (
Tubifex
) that burrow in O
2
-free sediments in pond or lake bottoms, for example, have been shown not only to survive but to feed, grow, and reproduce
while deprived of O
2
for 7 months!
Peak O
2
Consumption and Physical Performance at High Altitudes in Mountaineers Breathing Ambient Air
BOX 8.3
When people (and other mammals) are exposed to decreased atmospheric concentrations of O
2
, they marshal vigor-
ous physiological defenses, discussed in Boxes 23.2 and 24.5. Because of these responses, resting
or moderately active
people show a substantial degree of oxygen regulation as the ambient O
2
level falls with increasing altitude. Here, however, we consider the most demand-
ing of circumstances: the capability of mountaineers for all-out physical effort
at the highest altitudes on Earth.
As the figure shows, when people are asked to work hard enough that they take in O
2
at their peak rate, their maxi-
mum rate of O
2
consumption becomes a smaller and smaller fraction of their rate at sea level as altitude increases. The cost of any particular form of physical exertion remains the same regardless of altitude, however. Accordingly, a rate of climbing that is distinctly submaximal at low alti-
tudes can become maximal, or even su-
pramaximal, at high altitudes. At altitudes near the top of Mt. Everest (8848 m), the maximum rate of O
2
consumption is so low that even minimum rates of climbing require about the maximum
possible rate of O
2
consumption. Work that requires 100% of a person’s maximum rate of O
2
consumption is always extremely taxing: barely possible. When, in 1978, Reinhold Messner and Peter Habeler became the first to reach the summit of Mt. Everest without supplemental O
2
, they reported climbing so slowly near the top that, even though they felt they were working at their limits, they required an hour to cover the final 100 meters!
Box Extension 8.3
provides more infor-
mation on climbing Mt. Everest.
Maximum rate of O
2
consumption as percentage of rate at sea level (%)
Altitude (meters above sea level)
100
90
80
70
60
50
40
30
20
0
1000 2000
3000 4000 5000
6000 7000 8000 9000
Maximum rates of O
2
consumption of human mountaineers at increasing altitudes Values are expressed as percentages of the values at sea level. Mountain-
eers were breathing from the ambient air, not from O
2
tanks. (After Fulco et al. 1998.)
08_HILL4E.indd 210
4/1/16 1
Your preview ends here
Eager to read complete document? Join bartleby learn and gain access to the full version
- Access to all documents
- Unlimited textbook solutions
- 24/7 expert homework help
Anaerobes are rare among aquatic vertebrates. Nonetheless, two species of related cyprinid fish are known to have extraordinary abilities to live without O
2
. One of these is the common goldfish (
Carassius auratus
), which is reported to survive in O
2
-free water for 11–24 h at 20°C and for 1–6 days at 10°C. No wonder goldfish survive the tender loving care of 5-year-olds! The second species, which is even more tolerant of anoxia than the goldfish, is the crucian carp (
Carassius carassius
), a common inhabitant of northern European ponds. It can survive without O
2
for several months at temperatures below 10°C (see Figure 8.15)! The ability of crucian carp to live without O
2
permits them to evade predators by living in ponds that become O
2
depleted, where the predators die. Their physiology, therefore, is a key to their ecological success.
For both invertebrate and vertebrate aquatic anaerobes, metabolic depression (see Figure 8.14) is typically a key strategy used to survive anoxia. TABLE 8.2
presents data on metabolic depression in goldfish. Although their O
2
consumption falls to zero during anoxia, direct measurements of heat produc-
tion show that metabolism continues, but it does so at a highly depressed rate. Metabolic depression lowers ATP requirements and thus relaxes demands on the ATP-producing mechanisms that are available to make ATP in the absence of O
2
.
MECHANISMS OF INVERTEBRATE ANAEROBIOSIS The most common principal products of the anaerobic biochemical pathways used for ATP synthesis by invertebrate aquatic anaerobes are ace-
tic acid, succinic acid, propionic acid, and the amino acid alanine. These products obviously signal that the biochemical pathways of anaerobic catabolism in these animals differ from simple anaerobic glycolysis. The path-
ways are elaborate. They often permit the animals to catabolize anaerobically not only carbohydrates but also other classes of food molecules, notably amino acids, and they typically yield more ATP per food molecule than anaerobic glycolysis does. Invertebrate anaerobes often excrete their anaerobic end products or derivatives of them. Excretion wastes the energy value of the carbon compounds excreted, but it helps limit acidification of the body fluids and helps prevent self-limitation of the ATP-generating mechanisms, allowing the animals to sustain all their vital func-
tions in the absence of O
2
for protracted periods. MECHANISMS OF ANAEROBIOSIS IN GOLDFISH AND CRUCIAN CARP The goldfish and crucian carp provide a possibly amusing end to this chapter because they raise the question of whether certain animals are constantly drunk. Of all vertebrate animals, these fish are the most proficient known anaerobes because not only do they survive with-
out O
2
for long periods (see Figure 8.15), but also, unlike the turtles we discussed earlier, they remain conscious and capable of responding behaviorally to their environments.
The swimming muscles
of these fish possess biochemical spe-
cializations, including an unusual form of the enzyme alcohol dehydrogenase (ADH). This form of ADH strongly favors the formation of ethanol (ethyl alcohol) under prevailing tissue condi-
tions. During anoxia all the tissues of the fish, including the brain, synthesize ATP by anaerobic glycolysis and produce lactic acid. The swimming muscles convert this lactic acid (some of it brought to the muscles from the other tissues) to ethanol and CO
2
. The produc-
tion of ethanol does not increase ATP yield. Rather, its principal advantage is believed to be that it makes possible the excretion
of the carbon chains produced by anaerobic glycolysis. Unlike lactic acid, ethanol is lost across the gills into the water that the fish inhabit (
FIGURE 8.17
). This excretion limits end-product accumulation and TABLE 8.2 Average rates of O
2
consumption, heat production, and carbohydrate use in goldfish (
Carassius auratus
) before and during exposure to anoxia at 20°C
Note that although metabolic rate decreases in anoxia, the rate of use of carbohydrate stores increases because production of ATP is far less efficient by anaerobic glycolysis than by aerobic catabolism.
Property measured
a
At normal O
2
levels
After 3 h of anoxia
After 8 h of anoxia
Oxygen consumption (mmol/kg•h)
1.51
0
0
Heat production (J/ kg•h)
709
203
206
Carbohydrate catabolism (mg/kg•h)
43
221
234
Source:
van Waversveld et al. 1989.
a
All rates are expressed per kilogram of adjusted body weight. Adjusted weights were calculated in a way intended to remove allometric effects of different body sizes.
Blood vessel
Ethanol
Gills
Brain
Brain
Viscera
PDH
ADH
Pyruvic acid
Ethanol
LDH
Lactic acid
Enzyme
catalysts
Swimming
muscles
Swimming
muscles
Lactic acid
FIGURE 8.17 Excretion of the end product of anaerobic glycolysis as ethanol When crucian carp (
Carassius carassius
, shown here) and goldfish are living in O
2
-free water, all tissues—including the brain—make ATP by anaerobic glycolysis from stored gly-
cogen. In most fish, when lactic acid is made, it accumulates in the body. In the crucian carp and goldfish, however, the swimming muscles have an unusual ability to convert lactic acid to ethanol, which then is readily lost across the gills. In the swimming muscles, lactic acid is first converted to pyruvic acid, a reaction catalyzed by lactate dehydro-
genase (LDH). Pyruvic acid is then converted to acetaldehyde—catalyzed by pyruvate dehydrogenase (PDH)—and acetaldehyde is converted to ethanol—catalyzed by alcohol dehydrogenase (ADH). (After a concept by Bickler and Buck 2007.)
08_HILL4E.indd 211
4/1/16 1
Your preview ends here
Eager to read complete document? Join bartleby learn and gain access to the full version
- Access to all documents
- Unlimited textbook solutions
- 24/7 expert homework help
body-fluid acidification, thereby helping to prevent self-limitation of the ATP-generating mechanisms.
The brains of goldfish and crucian carp living in O
2
-free water exhibit far less metabolic depression than those of turtles. The more-limited metabolic depression permits the fish to remain conscious and responsive. It also increases demands for anaerobic ATP synthesis by brain cells. Crucian carp build up stores of brain glycogen with the approach of winter—the season when they are most likely to face anoxia. These stores provide fuel for making the ATP that keeps the brain functional.
The fish do not get drunk! Their rate of excretion of ethanol is great enough to keep their tissue concentrations of ethanol below the drunken level.
SUMMARY
Responses to Impaired O
2
Influx from the Environment
Many of the animals that are adapted to living without O
2
undergo metabolic depression when deprived of O
2
. Metabolic depression can be so profound as to lower an animal’s metabolic rate to less than 5% of the usual rate, thereby greatly reducing the rate at which ATP must be supplied by catabolic mechanisms.
Invertebrate anaerobes deprived of O
2
produce ATP by means of a diversity of complex anaerobic catabolic pathways that generate end products such as acetic acid, succinic acid, and propionic acid. The invertebrates commonly excrete these organic products during anoxia as a way of avoiding end-product accumulation in their bodies.
Virtually all vertebrates use simple anaerobic glycolysis to produce ATP in tissues deprived of O
2
, and vertebrates invariably retain lactic acid in their bodies, setting the stage for potential metabolic self-limitation. Usually when vertebrates experience anoxia, it is strictly regional; whereas some tissues become anoxic, others—most notably the CNS—retain an O
2
supply. Only a few vertebrates can tolerate total-body anoxia.
Turtles capable of total-body anoxia employ anaerobic glycolysis to make ATP. A key part of their strategy for survival is a metabolic depression of the CNS sufficiently profound to produce a comatose state.
Goldfish and crucian carp undergoing total-body anoxia remain alert. They have the unusual ability to convert lactic acid to ethanol, which they can excrete, thereby preventing end-product accumulation in their bodies.
STUDY QUESTIONS
1. One approach to conservation of fish populations is to release unwanted fish accidentally caught in trawling nets. Such fish often have very high concentrations of lactic acid in their bodies. Why do you think they have these high concentrations of lactic acid, and how might their survival after release be affected by their condition?
2. Explain how the reactions that produce and use ATP serve, together, as an energy shuttle mechanism in cells.
3. Explain the concept of redox balance. What conditions must exist, for example, for cytochrome oxidase to be in redox balance?
4. How does the reduction of pyruvic acid create a state of redox balance in anaerobic glycolysis?
5. Outline the chemiosmotic hypothesis for the mechanism by which oxidative phosphorylation is coupled with electron transport. How does uncoupling occur in tissues with uncoupling protein 1 (UCP1)? Under what circumstances would uncoupling be disadvantageous, and under what circumstances might it be advantageous?
6. Using two or three carefully chosen examples, illustrate the point that during physical activity, behavior and biochemistry are intimately linked, such that an animal’s exercise performance depends on the mechanisms that are making ATP for the exercise.
7. Assuming that an animal uses a catabolic pathway that produces organic products, such as lactic acid or propionic acid, compare the pros and cons of retaining or excreting the organic molecules.
8. Why is it important to distinguish temporary
electron (hydrogen) acceptors in cells from final
electron acceptors? What are the unique advantages of O
2
as an electron acceptor?
9. Why does an oxygen deficit occur at the start of submaximal exercise in vertebrates? What are the mechanisms of ATP production during the oxygen deficit phase, and how is ATP made in the ensuing pay-as-you-go phase?
10. A single individual can differ from time to time in his or her maximum rate of O
2
consumption. For example, athletic training in people can raise the maximum rate of O
2
consumption by 10%–30%, whereas going to high altitudes can lower it (see Box 8.3). Explain how these sorts of changes in the maximum rate of O
2
consumption can make a single type of exercise (such as jogging at 6 miles per hour) shift from being submaximal to supramaximal, or vice versa. What are the physiological implications of such shifts?
11. There has been a great deal of debate over whether the ratio of SO to FG fibers in the muscles of individual people or other animals is fixed genetically. Researchers have asked whether the ratio of fiber types can be altered during an individual’s lifetime by various sorts of training or other experiences. Why would a change in the ratio of fiber types be of interest and importance? Design experiments or other sorts of studies that would help elucidate whether the ratio of fiber types can undergo change.
Go to
sites.sinauer.com/animalphys4e for box extensions, quizzes, flashcards, and other resources.
REFERENCES
Alberts, B., A. Johnson, J. Lewis, and four additional authors. 2014. Molecular Biology of the Cell
, 6th ed. Garland, New York. A superior and time-proven presentation of all aspects of cellular physiology, including the catabolic mechanisms, emphasiz-
ing mammals. Excellent illustrations for understanding difficult concepts.
Åstrand, P.-O., K. Rodahl, H. A. Dahl, and S. B. Strømme. 2003. Textbook of Work Physiology: Physiological Bases of Exercise
, 4th ed. Human Kinetics, Champaign, IL. A classic treatment of the physiology of all forms of human work, from housework to Nordic skiing.
08_HILL4E.indd 212
4/1/16 1
Your preview ends here
Eager to read complete document? Join bartleby learn and gain access to the full version
- Access to all documents
- Unlimited textbook solutions
- 24/7 expert homework help
Recommended textbooks for you
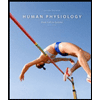
Human Physiology: From Cells to Systems (MindTap ...
Biology
ISBN:9781285866932
Author:Lauralee Sherwood
Publisher:Cengage Learning
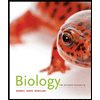
Biology: The Dynamic Science (MindTap Course List)
Biology
ISBN:9781305389892
Author:Peter J. Russell, Paul E. Hertz, Beverly McMillan
Publisher:Cengage Learning
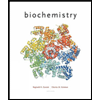
Biochemistry
Biochemistry
ISBN:9781305577206
Author:Reginald H. Garrett, Charles M. Grisham
Publisher:Cengage Learning
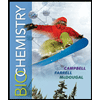
Biochemistry
Biochemistry
ISBN:9781305961135
Author:Mary K. Campbell, Shawn O. Farrell, Owen M. McDougal
Publisher:Cengage Learning
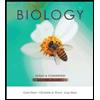
Biology Today and Tomorrow without Physiology (Mi...
Biology
ISBN:9781305117396
Author:Cecie Starr, Christine Evers, Lisa Starr
Publisher:Cengage Learning
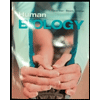
Human Biology (MindTap Course List)
Biology
ISBN:9781305112100
Author:Cecie Starr, Beverly McMillan
Publisher:Cengage Learning
Recommended textbooks for you
- Human Physiology: From Cells to Systems (MindTap ...BiologyISBN:9781285866932Author:Lauralee SherwoodPublisher:Cengage LearningBiology: The Dynamic Science (MindTap Course List)BiologyISBN:9781305389892Author:Peter J. Russell, Paul E. Hertz, Beverly McMillanPublisher:Cengage LearningBiochemistryBiochemistryISBN:9781305577206Author:Reginald H. Garrett, Charles M. GrishamPublisher:Cengage Learning
- BiochemistryBiochemistryISBN:9781305961135Author:Mary K. Campbell, Shawn O. Farrell, Owen M. McDougalPublisher:Cengage LearningBiology Today and Tomorrow without Physiology (Mi...BiologyISBN:9781305117396Author:Cecie Starr, Christine Evers, Lisa StarrPublisher:Cengage LearningHuman Biology (MindTap Course List)BiologyISBN:9781305112100Author:Cecie Starr, Beverly McMillanPublisher:Cengage Learning
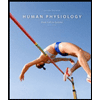
Human Physiology: From Cells to Systems (MindTap ...
Biology
ISBN:9781285866932
Author:Lauralee Sherwood
Publisher:Cengage Learning
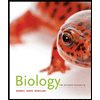
Biology: The Dynamic Science (MindTap Course List)
Biology
ISBN:9781305389892
Author:Peter J. Russell, Paul E. Hertz, Beverly McMillan
Publisher:Cengage Learning
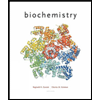
Biochemistry
Biochemistry
ISBN:9781305577206
Author:Reginald H. Garrett, Charles M. Grisham
Publisher:Cengage Learning
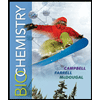
Biochemistry
Biochemistry
ISBN:9781305961135
Author:Mary K. Campbell, Shawn O. Farrell, Owen M. McDougal
Publisher:Cengage Learning
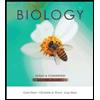
Biology Today and Tomorrow without Physiology (Mi...
Biology
ISBN:9781305117396
Author:Cecie Starr, Christine Evers, Lisa Starr
Publisher:Cengage Learning
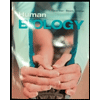
Human Biology (MindTap Course List)
Biology
ISBN:9781305112100
Author:Cecie Starr, Beverly McMillan
Publisher:Cengage Learning